5 Facts We Can Learn If LIGO Detects Merging Neutron Stars
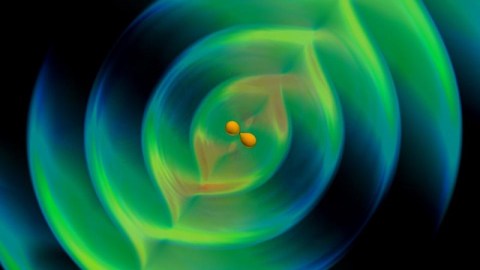
Are we about to make a breakthrough to go beyond black holes? Here’s what it means if we do!
“It’s becoming clear that in a sense the cosmos provides the only laboratory where sufficiently extreme conditions are ever achieved to test new ideas on particle physics. The energies in the Big Bang were far higher than we can ever achieve on Earth. So by looking at evidence for the Big Bang, and by studying things like neutron stars, we are in effect learning something about fundamental physics.” –Martin Rees
If there’s one major difference between General Relativity and Newtonian gravity, it’s this: in Einstein’s theory, nothing lasts forever. Even if you had two perfectly stable masses in orbit around one another — masses that never burned out, lost material, or otherwise changed — their orbits would eventually decay. Whereas in Newtonian gravity, two masses would orbit their mutual center of gravity for an eternity, relativity tells us that a tiny amount of energy gets lost with every moment that one mass is accelerated by the gravitational field it passes through. That energy doesn’t disappear, but gets carried away in the form of gravitational waves. Over long enough time periods, enough energy is radiated away that those two orbiting masses will touch and merge together. Three times, now, LIGO has seen this happen for black holes. But it may be about to take the next step, and see neutron stars merge for the first time.
Any masses caught in this gravitational dance will emit gravitational waves, causing their orbits to decay. The reason LIGO has detected black holes merging are threefold:
- They’re incredibly massive,
- They’re the most compact objects in the Universe,
- And they orbit with the right frequency, in the final merger stages, to be detectable by LIGO’s laser arms.
That combination — large masses, short distances, and the right frequency range — gives the LIGO team a huge search area over which they’re sensitive to merging black holes. Out to many billions of light years away, the ripples from these massive inspirals can be felt even here on Earth.
The Universe has many other objects of interest that produce large-magnitude gravitational waves. Supermassive black holes at the centers of galaxies swallow gas clouds, planets, asteroids, and even other stars and black holes all the time. Unfortunately, since the event horizons are so much larger, they take too long to orbit and occur in the wrong frequency range for LIGO to see them. White dwarfs, binary stars, and other planetary systems suffer the same problem: these objects are physically too big, and therefore take a long time to orbit. They all take so long, in fact, that we’d need a space-based gravitational wave observatory — like LISA — to see them. But there’s another hope for LIGO that has that same combination (massive, compact, right frequency) to be seen: merging neutron stars.
Neutron stars may not be as massive as black holes, but they can likely be up to two or three times the mass of the Sun: about 10–20% the mass of previously detected LIGO events. They’re almost as compact as black holes, having a physical size that’s only ten kilometers in radius or so. Even though black holes collapse down to a singularity, they still have an event horizon, and a neutron star’s physical size (it’s basically just a giant atomic nucleus) is barely bigger than a black hole’s event horizon size. And their frequency, particularly in the last few seconds of a merger, lines up very, very well with what LIGO is sensitive to. If an event goes off in the right place, here are five incredible facts that we could learn.
1.) Do merging neutron stars really create gamma-ray bursts? There’s an incredible idea out there: that short gamma-ray bursts, which are incredibly energetic but last for less than two seconds, are caused by merging neutron stars. They occur in old galaxies in regions that aren’t forming new stars, suggesting that only stellar corpses could explain them. But until we can know what led up to a short gamma-ray burst, we can’t be sure what caused them. If LIGO can detect a merging neutron star pair in gravitational waves, and we can then see a short gamma-ray burst immediately after, this could finally verify and validate one of the most interesting ideas in astrophysics.
2.) When neutron stars collide, how much of their mass doesn’t become a black hole? When you look at the heavier elements in the periodic table and ask how they were made, you probably think “supernovae” is the answer. After all, that’s normally the story that astronomers tell, and it’s partially true. But the majority of the heaviest elements in the periodic table — mercury, gold, tungsten, lead, etc. — are actually made from neutron star collisions. Most of the mass, somewhere around 90–95%, from the neutron stars goes into forming a single black hole at the center, but the remaining outer layers are ejected, forming the majority of these elements in our galaxy. (Note: if the combined mass of the two merging neutron stars is below a certain threshold, they’ll form a central neutron star instead of a black hole. This should be rare, but not impossible.) Exactly how much gets ejected? If LIGO detects such an event, it should tell us.
3.) How far out can LIGO see merging neutron stars? This isn’t a question about the Universe itself, but rather about how close to (or, conceivably, in excess of) design sensitivity advanced LIGO has gotten. For light, if an object is 10 times farther away, it’s only 1/100th as bright; but for gravitational waves, an object 10 times as distant has a gravitational wave signal that’s still 1/10th as strong. Black holes might be observable to LIGO at a distance of many millions of light years, but neutron stars might only be visible if they merge in a handful of our closest large galaxy clusters. If we see one, we can truly know just how good our equipment is… and how good it needs to be.
4.) What sort of afterglow do merging neutron stars leave? We know, in a few cases, that strong events consistent with neutron star collisions have occurred, and that they sometimes leave signatures in other electromagnetic bands. Not only should there be a reasonable chance of gamma rays, but there may even be a UV, optical, infrared, or radio counterpart. Or, perhaps, there will be a multispectral counterpart, appearing in all five such bands, in that order. With a neutron star merger occurring so close (that LIGO could detect it), we might have a real opportunity to get in on the ground floor of one of nature’s most incredible observations.
And the biggest one of all…
5.) For the first time, we could combine gravitational wave astronomy with traditional (light-based) astronomy. The previous LIGO events were spectacular, but there was no way to see the mergers through a telescope. After all, the whole scenario had two strikes working against it:
- Event positions cannot be accurately be determined from only two detectors, even in principle, and
- Black hole mergers aren’t thought to have a bright electromagnetic (light-based) counterpart.
Now that VIRGO is operational and synced with the twin LIGO detectors, we can make much improved determinations of where in space a gravitational wave event occurred. But more importantly, because neutron star mergers should have an electromagnetic counterpart, this could mark the very first time that gravitational wave astronomy and traditional astronomy can be used to observe the same event in the Universe!
We have already entered a new age in astronomy, where we’re not just using telescopes, but interferometers. We’re not just using light, but gravitational waves, to view and understand the Universe. If merging neutron stars reveal themselves to LIGO, even if the events are rare and the detection rate is low, it means we’ll have crossed that next frontier. The gravitational sky and the light-based sky will no longer be strangers to one another. Instead, we’ll be one step closer to understanding how the most extreme objects in the Universe actually work, and we’ll have a window into our cosmos that no human has ever had before.
Ethan Siegel is the author of Beyond the Galaxy and Treknology. You can pre-order his third book, currently in development: the Encyclopaedia Cosmologica.