Can We Test Gravitational Waves For Wave-Particle Duality?
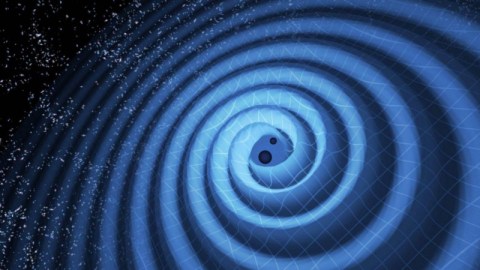
If quantum gravity is right, these gravitational ripples must be more than waves; they must be particles, too.
Back in February of 2016, LIGO made an announcement that changed our picture of the Universe forever: from more than a billion light-years away, two massive black holes, of 36 and 29 solar masses, had inspiraled and merged. The result of that merger was a single black hole of 62 solar masses, with the remaining 3 solar masses converted into pure energy via Einstein’s E = mc², rippling throughout the Universe in the form of gravitational waves.
Since that time, LIGO has risen into the double digits with the number of detections it’s made, as gravitational waves are now undoubtedly real and teaching us an incredible amount about our Universe. But all of this is still information about our Universe according to our classical theory of gravity: General Relativity. If quantum physics is right, then wave-particle duality is real, even for gravitational waves. Here’s what that means.
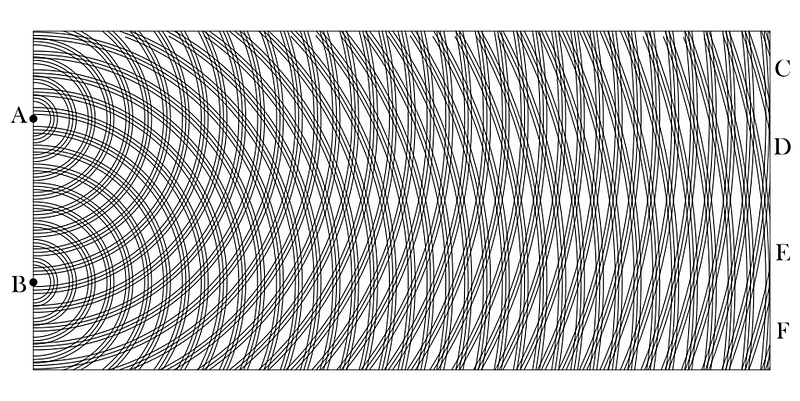
It’s no stretch to claim that wave-particle duality is one of the strangest quantum phenomena ever uncovered. It started out simply enough: matter was made of particles, things like atoms and their constituents, and radiation was made of waves. You could tell something was a particle because it would do things like collide and bounce off of other particles, stick together, exchange energy, become bound, etc.
Similarly, you could tell something was a wave because it would diffract and interfere with itself. Newton got this one wrong about light, thinking it was made of particles, but others such as Huygens (his contemporary) and then the early-1800s scientists like Young and Fresnel showed definitively that light exhibited properties that couldn’t be explained without considering it a wave.
The most obvious phenomena appear when you pass light through a double slit: the pattern that shows up on a background screen shows that the light interferes both constructively (leading to bright spots) and destructively (leading to dark spots).
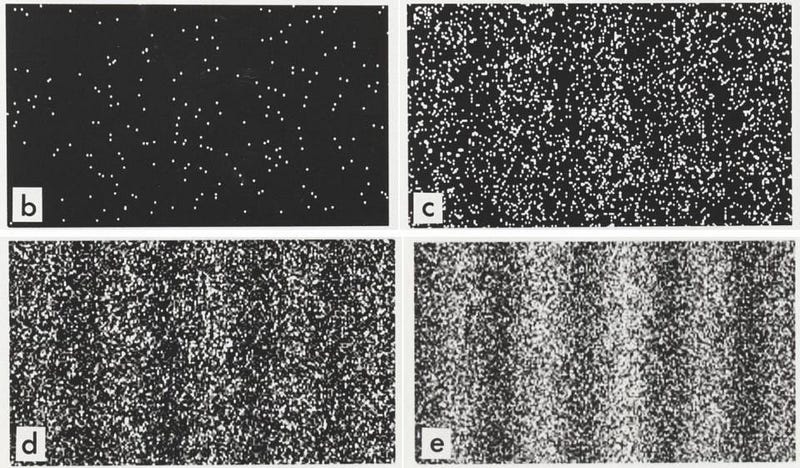
This phenomenon, of interference, is uniquely a product of waves. The double slit experiment, and subsequent, more sophisticated analogues, established that light was a wave. But this got more confusing in the early 1900s, with the discovery of the photoelectric effect. When you shone light on a certain material, occasionally electrons would get “kicked off” by the light.
If you made the light redder (and hence, lower energy) — even if you made the light arbitrarily intense — the light wouldn’t kick off any electrons. But if you kept the bluer (and hence, higher energy) light, even if you turned the intensity way, way down, you’d still kick off electrons. Shortly thereafter, we were able to discover that light is quantized into photons, and that even individual photons could act like particles, ionizing the electrons if they were of the right energy.
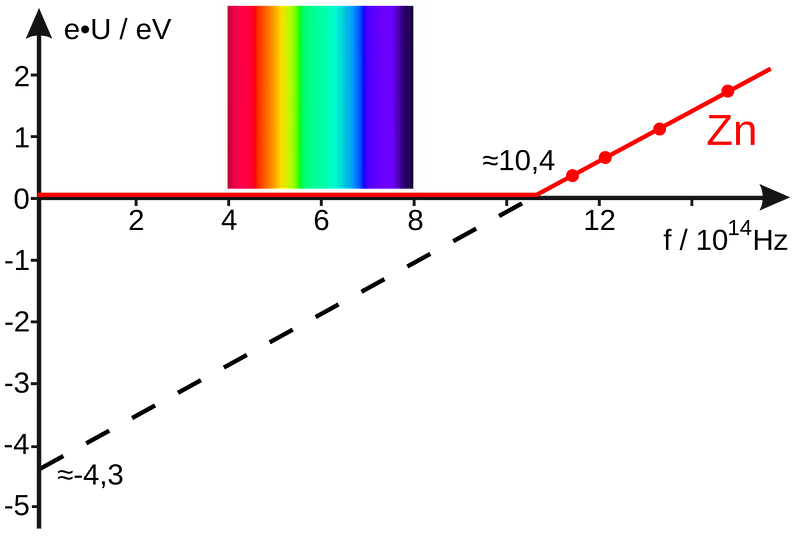
Even stranger realizations came in the 20th century, as we discovered that:
- Single photons, when you passed them through a double slit one-at-a-time, would still interfere with themselves, producing a pattern consistent with a wave nature.
- Electrons, known to be particles, exhibited this interference and diffraction pattern as well.
- If you measured which slit a photon or electron goes through, you don’t get an interference pattern, but if you don’t measure it, you do get one.
It seems that every particle we’ve ever observed can be described as both a wave and a particle. Moreover, quantum physics teaches us that we need to treat it as both under the proper circumstances, or we won’t get the outcomes that agree with our experiments.
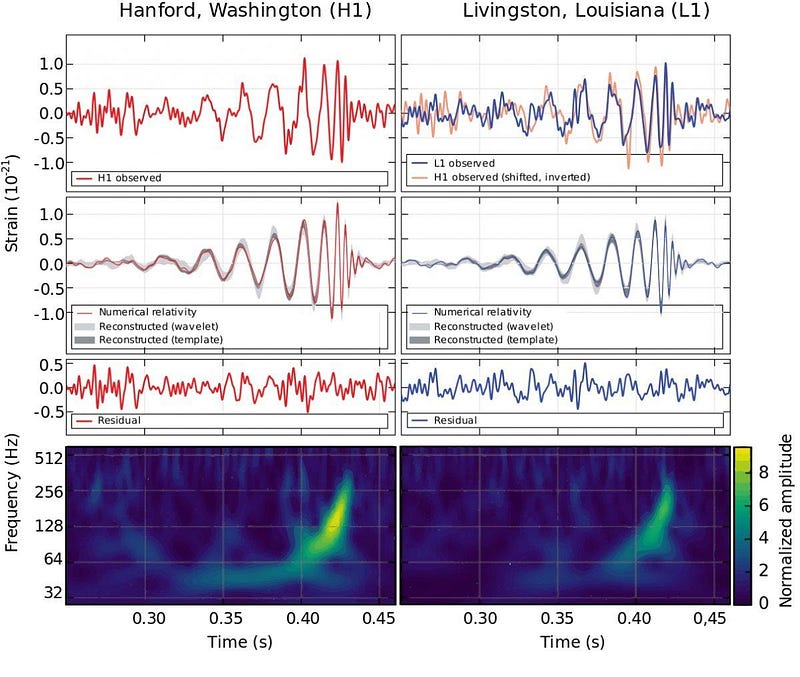
Now, at last, we’re ready to consider gravitational waves. These are sort of unique as far as physics is concerned, because we’ve only seen the wave-like part of them, never the particle-based part.
However, just like water waves are waves that are made of particles, we fully expect that gravitational waves are made of particles, too. Those particles ought to be gravitons (instead of water molecules), the particle that mediates the force of gravity under all known ideas that can give you a quantum theory of gravity. Gravitons are fully expected to emerge as a consequence of gravity being an inherently quantum force in nature, and gravitational waves ought to be made out of them.
Because it’s a wave, and because that wave has been observed to behave exactly as General Relativity predicts, including:
- during the inspiral phase,
- during the merger phase, and
- during the ringdown phase,
we can safely infer that it will continue to do all the wave-like things that General Relativity predicts. They’re a little different in detail than the other waves we’re used to: they’re not scalar waves like water waves, nor are they even vector waves like light, where you have in-phase, oscillating electric and magnetic fields.
Instead, these are tensor waves, which causes space to contract and rarify in perpendicular directions as the wave passes through that area.
These waves do a lot of the same things you’d expect from any sort of wave, including that
- they propagate at a specific speed through their medium (the speed of light, through the fabric of space itself),
- they interfere with any other ripples in space both constructively and destructively,
- these waves “ride” on top of whatever other spacetime curvature is already present,
- and if there were some way to cause these waves to diffract — perhaps by traveling around a strong gravitational source like a black hole — they would do exactly that.
In addition, as the Universe expands, we know these waves will do what all waves in the expanding Universe do: to stretch and expand as the background space of the Universe expands, too.
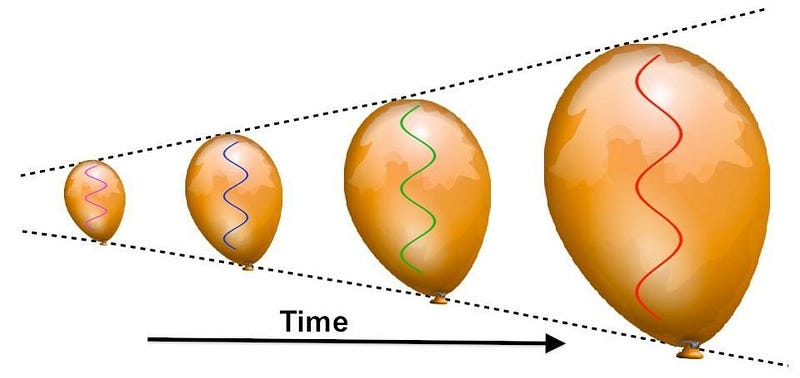
So the real question is, then, how do we test the quantum part of this? How do we look for the “particle” nature of a gravitational wave? In theory, a gravitational wave is similar to the earlier image that shows an apparent wave arising from many particles that move around: those particles are the gravitons and the overall apparent wave is what LIGO detected. There’s every reason to expect we’ve got a series of gravitons on our hands, that are:
- spin-2 particles,
- that are massless,
- that propagate at the speed of light,
- and that only interact through the gravitational force.
The constraints from LIGO on the second one — the masslessness — are extremely good: if the graviton does have a mass, it’s less than 1.6 x 10^-22 eV/c², or some ~10²⁸ times lighter than the electron. But until we figure out a way to test quantum gravity using gravitational waves, we won’t know whether the “particle” part of wave-particle duality holds for gravitons.
We’ve actually got a few chances for this, although LIGO is unlikely to succeed at any of them. You see, quantum gravitational effects are strongest and most pronounced where you have strong gravitational fields in play at very tiny distances. What better tool could there possibly be to probe this regime than merging black holes?
When two singularities merge together, these quantum effects — which should be departures from General Relativity — will show up at the moment of the merger, and just before (at the end of the inspiral) and just after (at the start of the ringdown) phases. Realistically, we’re looking at probing picosecond timescales rather than the micro-to-millisecond timescales LIGO is sensitive to, but this might not be impossible.
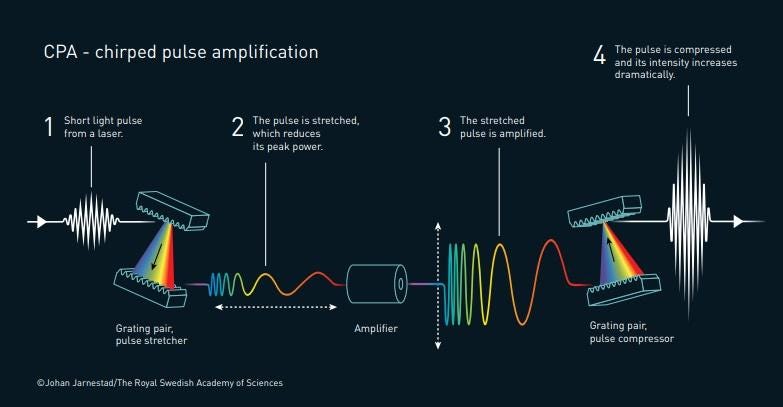
We’ve developed laser pulses that work in the femtosecond or even attosecond (10^-15 s to 10^-18 s) time ranges, and so it’s conceivable that we could be sensitive to tiny departures from relativity if we have enough of these interferometers going at once. It would take a tremendous leap in technology, including a large number of interferometers, and a significant reduction in noise and increase in sensitivity. But it’s not technically impossible; it’s just technologically difficult!
For a little more information, I once gave a video talk on gravitational waves, LIGO and what we learned from it to the Lowbrow Astronomers at the University of Michigan, and the full talk is currently online, with the last question touching on exactly this point.
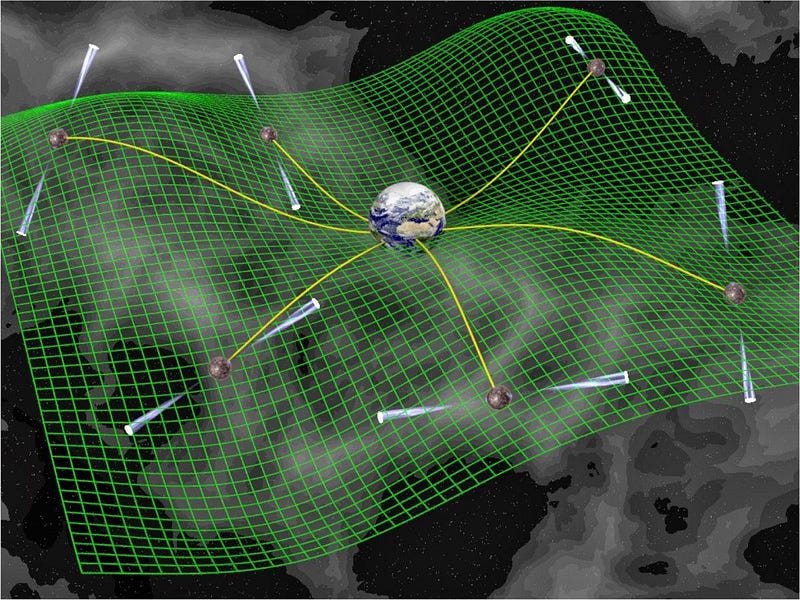
Although we have every reason to believe that gravitational waves are simply the quantum analog of electromagnetic waves, we have, unlike the electromagnetic photon, not yet risen to the technological challenges of directly detecting the gravitational particle that’s the counterpart of gravitational waves: the graviton.
Theorists are still calculating the uniquely quantum effects that should arise and are working together with experimentalists to design tabletop tests of quantum gravity, all while gravitational wave astronomers puzzle over how a future-generation detector might some day reveal the quantum nature of these waves. Although we expect gravitational waves to exhibit wave-particle duality, until we detect it, we cannot know for certain. Here’s hoping that our curiosity compels us to invest in it, that nature cooperates, and that we find out the answer once and for all!
Ethan Siegel is the author of Beyond the Galaxy and Treknology. You can pre-order his third book, currently in development: the Encyclopaedia Cosmologica.