What was it like when the first elements formed?
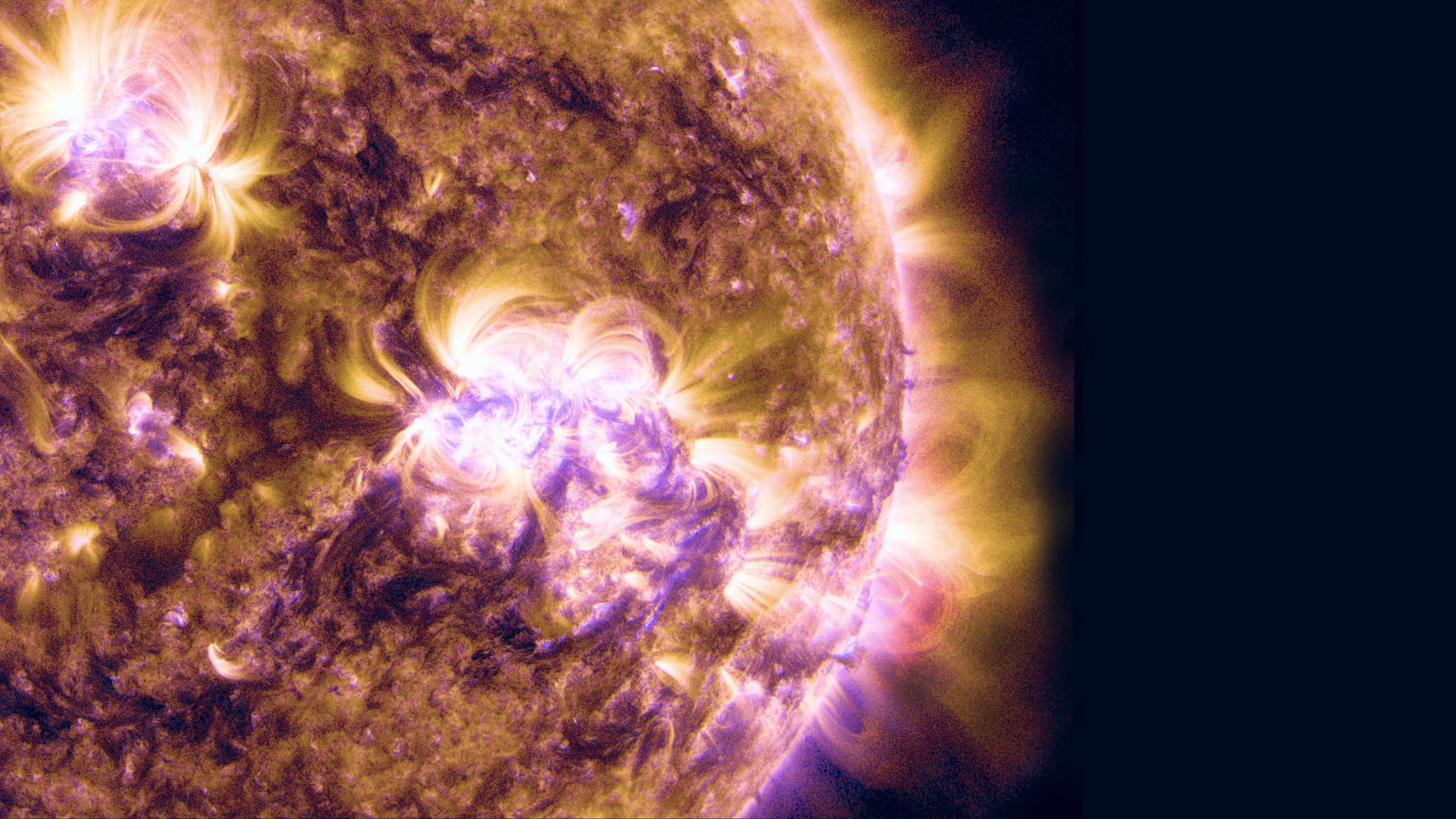
- Back in the earliest stages of the hot Big Bang, there were no elements at all: just a “soup” of free quarks and gluons and first, and then free protons and neutrons a little bit later.
- Yet, by the time the first stars formed, the Universe was made of ~75% hydrogen, ~25% helium, and a tiny, tiny amount of lithium: elements that weren’t present at the very beginning.
- Although the seeds were in place to form elements just seconds after the Big Bang, creating those elements is a process that takes minutes to begin, but decades to complete. Here’s why.
One of the most remarkable achievements in all of human history is the discovery of the scientific story of how our Universe began, evolved over time, and came to be the way it is today. Back in the very early stages of the Universe, we experienced conditions known as the hot Big Bang: where everything was extremely dense, energetic, and rapidly expanding. In these early stages, there were no bound states — no atoms, no atomic nuclei, not even protons and neutrons — just a free, hot plasma of particles and antiparticles. As the Universe expands, however, it cools, and numerous things ensue from that, including:
- matter winning out over antimatter,
- the electroweak symmetry breaking and the Higgs giving mass to the Universe,
- the formation of protons and neutrons,
- and the annihilation of the last of our cosmos’s antimatter.
By the time the Universe is 3 seconds old, there are no more free quarks; there is no more antimatter; neutrinos no longer collide with or interact with any of the remaining particles. We have more matter than antimatter, more than a billion photons for every proton or neutron, a ratio of about 85% protons to 15% neutrons, all while the Universe has cooled to now be just a little bit under ~10 billion K in temperature. But despite all of that cosmic evolution in only a few seconds, atomic nuclei — the determining factor in what element you are — cannot yet form. Here’s how that key step in our history occurs.

A whole slew of things happened during the first 3 seconds of the Universe’s history after the start of the hot Big Bang, but one of the last things to happen is most important for what comes next. The Universe, early on, was filled with protons and neutrons, which would — at high enough energies — collide with electrons or neutrinos to interconvert, or switch, from one type to the other. These reactions all conserved a quantum property known as “baryon number” (the total number of protons and neutrons) as well as electric charge, meaning that this phase began with a 50/50 split between protons and neutrons, with exactly enough electrons to balance the number of protons. This was the situation when the Universe was a few microseconds old.
But things won’t remain evenly split for long for an important reason: the neutron is more massive than the proton. It requires more energy, via Einstein’s E = mc², to create a neutron (and a neutrino) from a proton (and an electron) than for the reverse reaction to occur. As a result, as the Universe cools, more neutrons turn into protons than the other way around. By the time all is said and done, and a full ~3 seconds have elapsed since the start of the hot Big Bang, the Universe is 85-86% protons (with an equal number of electrons) and just 14-15% neutrons.
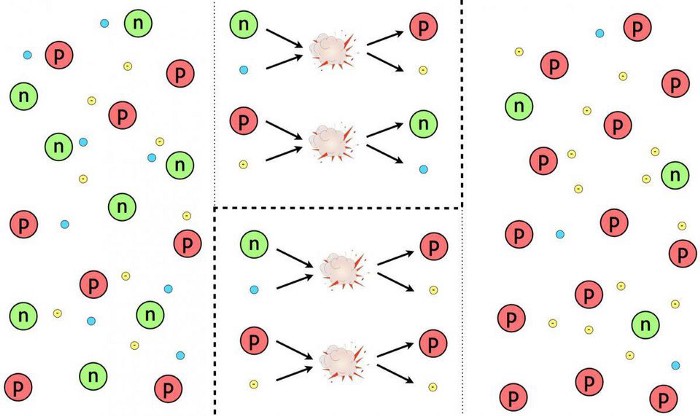
With protons, neutrons, and electrons all flying around under extremely hot, dense conditions, you envision conditions similar to something like what occurs in the center of our Sun: an actual nuclear fusion reactor. It seems so reasonable to think about the process of:
- protons and neutrons fusing together,
- building up heavier and heavier elements as they climb the periodic table,
- and giving off energy via Einstein’s E = mc² as these fusion reactions occur,
as the reactions that build bound elements out of raw protons (or raw protons and neutrons) must inevitably do.
Once you have atomic nuclei, you can envision that at some key time after that, the Universe will cool enough to enable electrons to bind to those nuclei, producing the full gamut of stable, neutral elements found in the periodic table today. After all, we see these elements everywhere we look: not only in the Sun, but within each and every star (and galaxy) ever discovered. It’s a reasonable line of thought, because these elements had to come from somewhere.

So why not right from the start: in the aftermath of the hot Big Bang?
It’s a great thought, and it’s a plausible pathway, but it’s not the one that reality actually takes. The odd thing is this: those heavy elements really do come from somewhere, but almost all of them are not from the Big Bang. No less an authority than George Gamow — the founder of the Big Bang theory — claimed that this hot, dense crucible was the perfect spot to form these elements.
Gamow was mistaken, however. The Universe does form elements during the hot Big Bang, but only a very select few.
There’s a reason for this that Gamow never anticipated, and that most of us may not have thought of at first glance, either. You see, in order to make elements, you need enough energy to fuse them together. But in order to keep them around and build heavier things out of them, you must make sure you don’t destroy them. And this is where the early Universe, in the aftermath of the hot Big Bang, lets us down.
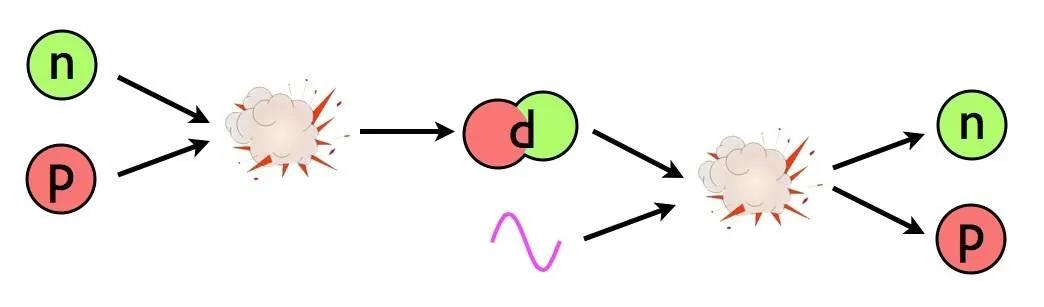
Let’s paint a (simplified) picture for you of what the early Universe was like when it was only a few seconds after the hot Big Bang began. At three seconds of age, we can treat the Universe as though it’s filled with:
- 85% protons (and an equal number of electrons),
- 15% neutrons,
- and about 1-to-2 billion photons for every proton or neutron.
(Yes, there are also neutrinos and antineutrinos, whatever dark matter is, and whatever dark energy is; they’re all present. They just aren’t relevant to this portion of the story.) In order to build a heavy element, the first step must be to either collide a proton with a neutron or a proton with another proton. The first step toward building anything more complicated out of the basic building blocks of atoms is to create a nucleus with two nucleons (like a proton and neutron) bound together.
This part is easy! The Universe makes deuterium nuclei, abundantly, with no problem. Proton-neutron collisions easily create the more stable deuterium, and even give off a high-energy photon of about ~2.2 MeV of energy in the process. Making deuterium is easy. The problem is that the instant we make it, it’s immediately destroyed.
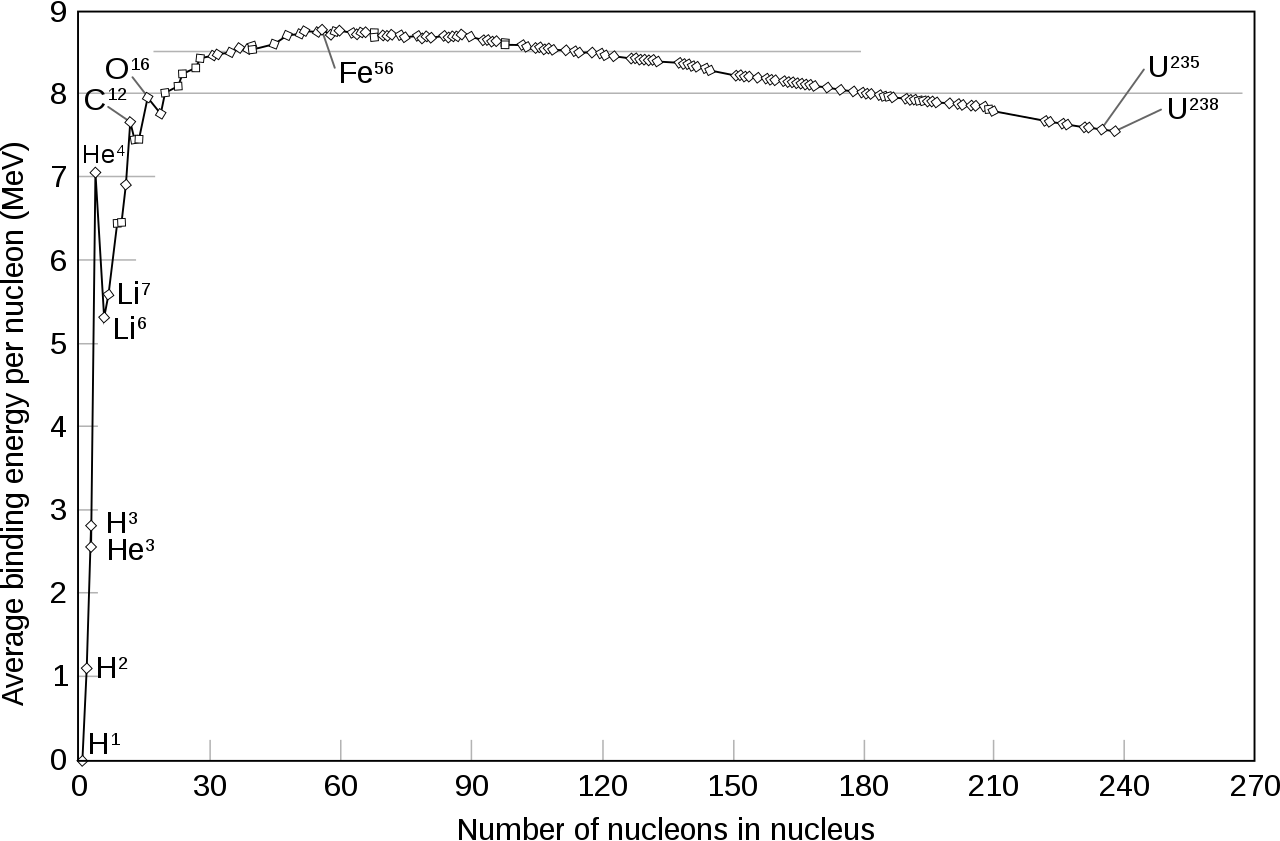
Let’s walk through the reason why. In a hot, dense Universe, where photons far outnumber protons and neutrons, the overwhelming odds are that as soon as you make a deuterium nucleus, the very next thing to collide with your deuteron will be a photon. (The odds, after all, are around 1-in-a-billion that it won’t be a photon!) At the extremely high energies found in the early stages of the hot Big Bang — remember, the Universe is at a temperature measured in the billions of degrees at this time — those photons have more than enough energy to immediately blast that deuteron back apart into a proton and neutron.
Even though a deuteron is less massive by about 2.2 MeV (mega-electron volts) than an individual, free proton or neutron, there are great numbers of photons present that are energetic enough to overcome that mass difference. Unfortunately for the Universe, Einstein’s E = mc², the very same equation that allows you to build up heavy elements through the process of nuclear fusion, can also prevent you from building what you want, too. For every reaction that occurs, after all, the reverse reaction is also possible.

From the time that protons and neutrons are first formed, deuterium is constantly being created. However, just as quickly as the Universe can make it, it’s also being destroyed at the same rate. Without that key “first step” on our elemental staircase in place, we can go no farther. As long as the Universe is this hot, there is nothing we can do but wait. Without a stable nucleus that’s got at least two nucleons (a proton and/or neutron) within it, you can’t build your way, one additional proton-or-neutron at a time, toward anything heavier.
For this reason, cosmologists call this epoch in our cosmic history the deuterium bottleneck: we would love to build heavier elements and we have the material to do so, but we must pass through an era where deuterium is so easily destroyed. This takes time, as even though the Universe cools as it expands, there are still enough photons around with sufficiently high energies to blast each created deuterium nucleus apart.
So we wait. We wait for the Universe to cool, which means it has to expand, stretching the photons’ wavelengths, until they fall below the critical threshold needed to break deuterium nuclei apart. But this takes more than three minutes to happen, and in the meantime, something else takes place. The unbound neutrons, so long as they’re free, are unstable, and start to radioactively decay.
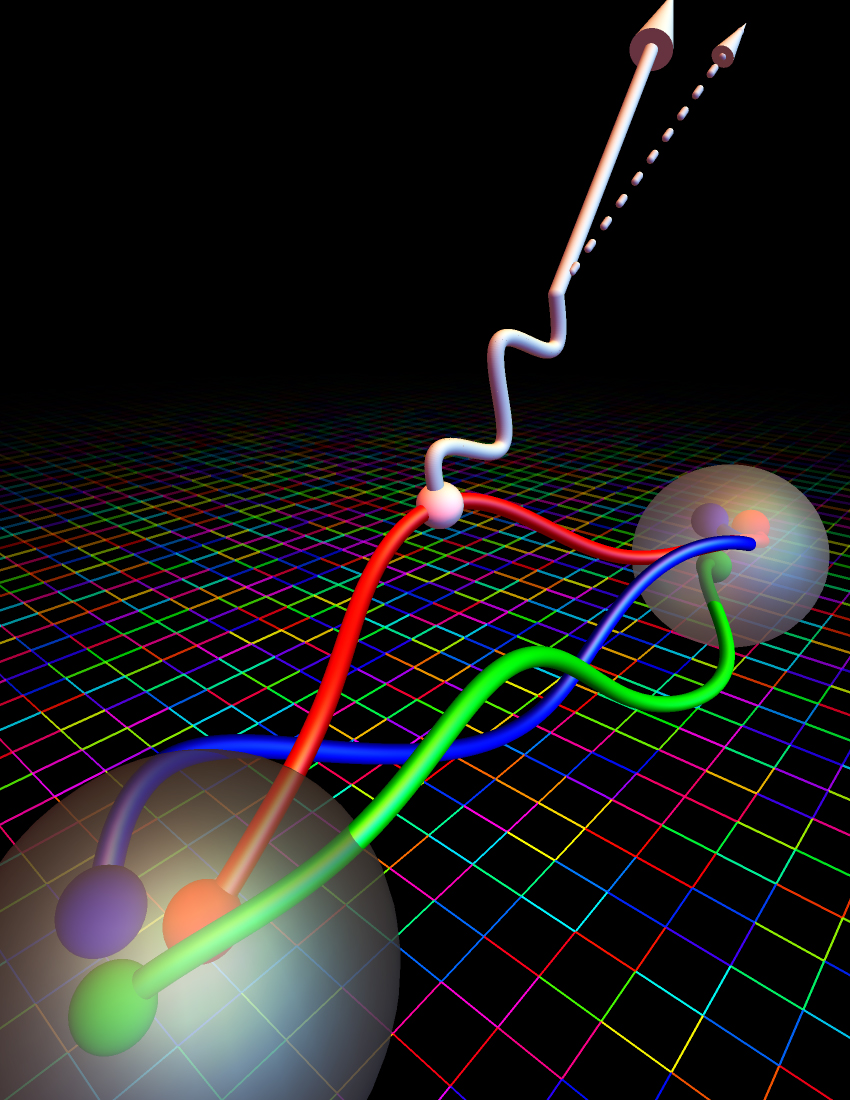
All radioactive elements have a certain probability of decaying in a certain amount of time, and we normally define that decay timescale by the term “half-life.” After one half-life, 50% of the original sample will have decayed away; after two half-lives, 75% decays away; after three half-lives, 87.5% decays away, etc. It turns out that neutrons, like all particles, have the same half-life today as they did early on in the Universe’s history; the laws of nature do not show any evidence of changing over time.
As we measure it today, a free neutron has a half-life of about 10.3 minutes. This means that if we wait around long enough, every neutron that we have will decay into a proton, an electron, and an anti-electron neutrino. In terms of an equation, it looks like this:
- n → p + e– + νe.
The actual time it takes for the Universe to expand-and-cool to the point where deuterium isn’t immediately blasted apart is about 3.5 minutes; enough time so that about 20% of the neutrons present will have decayed into protons over this timespan. What was a 50/50 split between protons and neutrons in the early stages became an 85/15 split after 3 seconds, and has now, after more than three minutes of radioactive decay, become more like 87.6% protons and 12.4% neutrons.
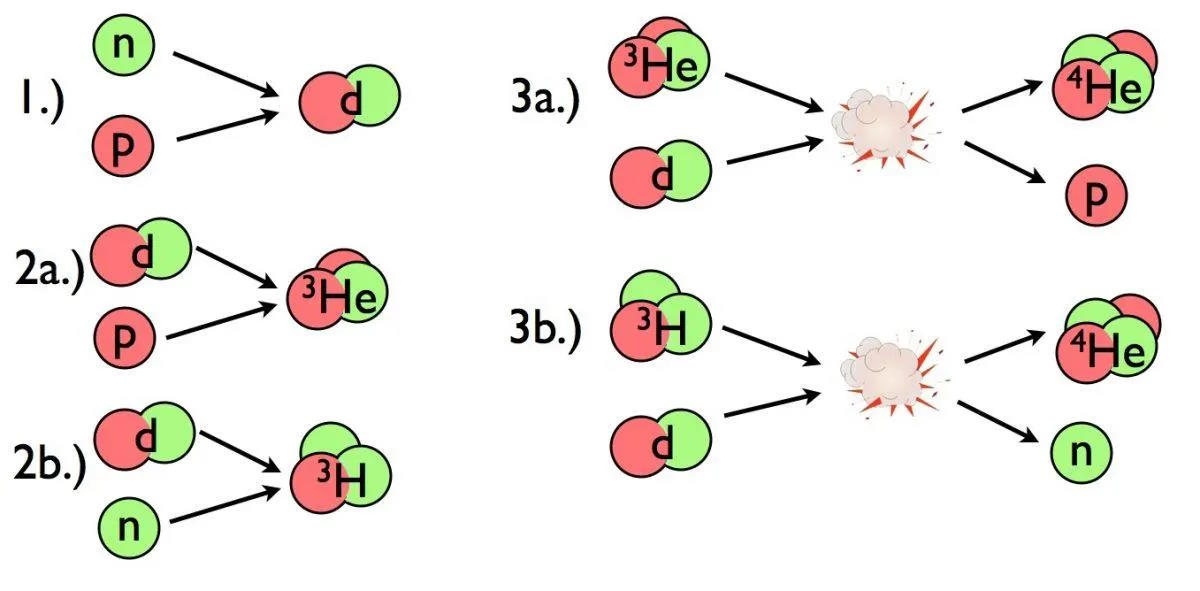
But now, the fun can truly begin. After somewhere between 3-and-4 minutes has elapsed since the start of the hot Big Bang, the Universe is cool enough that we can not only build deuterium, but build up and up the periodic table from there.
- Add another proton to a deuteron and you get helium-3, or alternatively, add another neutron to a deuteron and you get hydrogen-3, better known as tritium.
- If you then add another deuteron to either helium-3 or tritium, you get helium-4 out, plus either a proton or neutron, respectively.
Helium-4 is very stable; if you can reach this element, it’s extraordinarily difficult to blast apart. (It’s much more stable than deuterium.) By the time the Universe is 3 minutes and 45 seconds old, practically all of the neutrons have been used to form helium-4. In fact, if you were now to measure the various elements by mass, what you’d find is that the atomic nuclei are about:
- 75.2% hydrogen (protons),
- 24.8% helium-4 (2 protons and 2 neutrons),
- 0.01% deuterium (1 proton and 1 neutron),
- 0.003% tritium and helium-3 combined (tritium is unstable and will decay to helium-3, with 2 protons and 1 neutron, on timescales of decades), and
- 0.00000006% lithium-7 and beryllium-7 combined (where beryllium-7 is unstable and will decay into lithium-7 on timescales of several months).
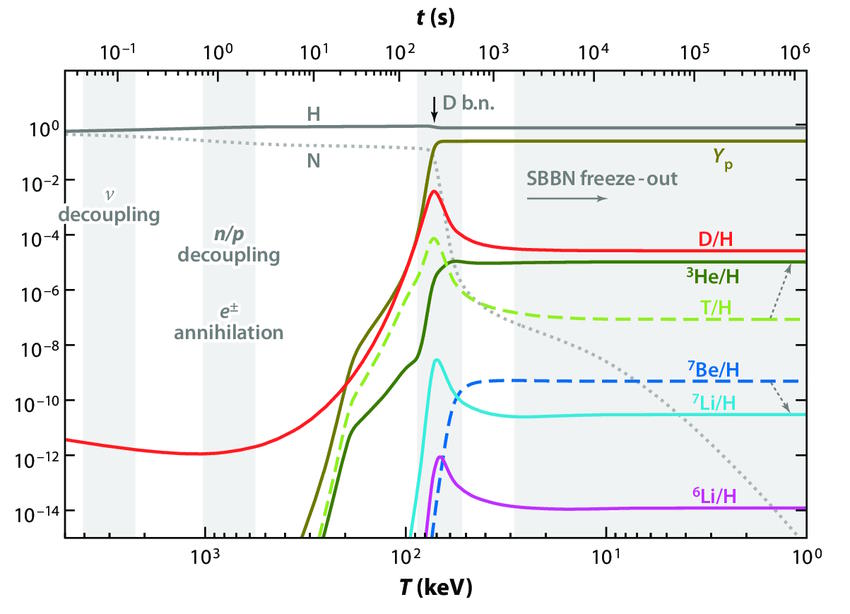
But that, unfortunately, is the end of the line for nuclear fusion occurring during the hot Big Bang. The big problem is that by this time, the Universe has expanded and cooled enough that its density is tiny: only one-billionth the density found in the Sun’s core. Nuclear fusion cannot occur any longer, as there are no ways to stable fuse either:
- a proton with helium-4 into lithium-5,
- or two helium-4 nuclei into beryllium-8.
These elements, Li-5 and Be-8, do exist, but both are highly unstable, and decay away after a tiny fraction of a second: less than a femtosecond, which isn’t enough time for another particle to get in and build up to even heavier, more stable elements. As a result, that’s all we get that’s forged in the furnace of the hot Big Bang: hydrogen and its stable isotopes, helium and its stable isotopes, and a teeny, tiny bit of lithium.
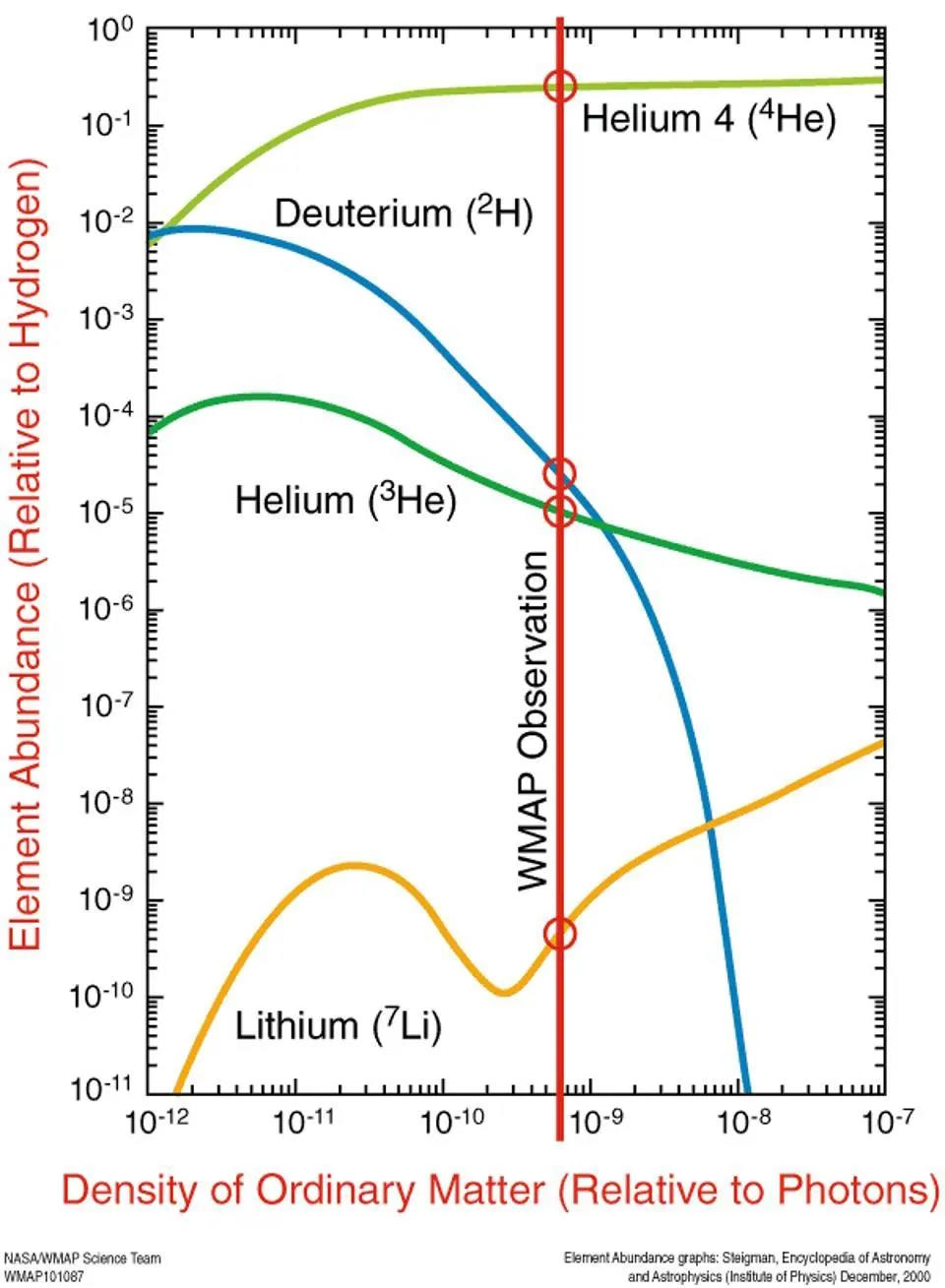
The Universe does form elements immediately after the Big Bang, but almost all of what it forms is either hydrogen or helium. There’s a tiny, tiny amount of lithium left over from the Big Bang, but it’s only around 1-part-in-a-billion by mass. Once the Universe cools down enough that electrons can bind to these nuclei, we’ll have our first elements: the ingredients that the very first generations of stars will be made out of.
But they won’t be made out of the elements we think of as essential to existence, including carbon, nitrogen, oxygen, silicon, phosphorus and more. Instead, it’s just hydrogen and helium, to the 99.9999999% level. It took less than four minutes to go from the start of the hot Big Bang to the first stable atomic nuclei, all amidst a bath of hot, dense, expanding-and-cooling radiation. The cosmic story that would lead to us has, in truth, finally begun.