How heavy hydrogen reveals the dark Universe
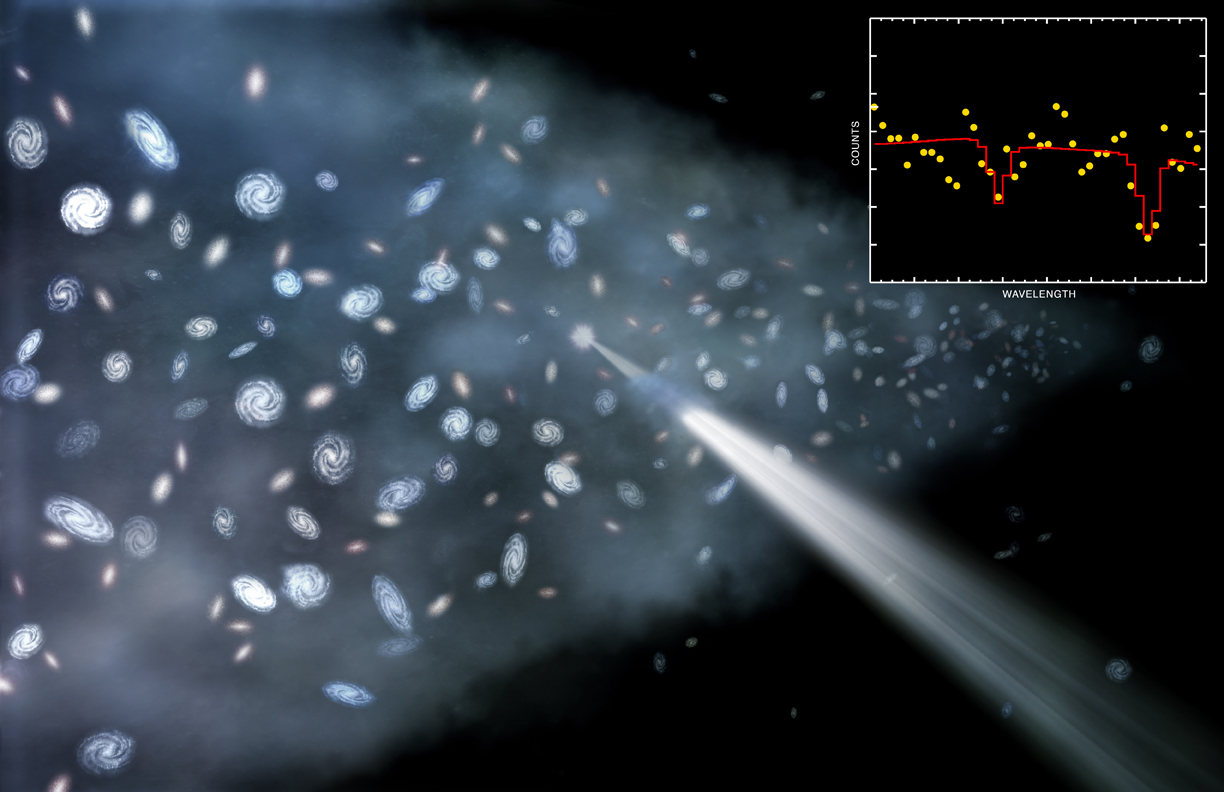
- In our Universe, we assert that only 5% of it is made out of normal matter: particles contained within the Standard Model. The remaining 95% is a mix of dark matter and dark energy.
- Typically, the best evidence for this picture comes from three independent sets of measurements: type Ia supernovae, the cosmic microwave background, and our cosmic large-scale structure.
- But even if you ignored all of that, you could still determine that only 5% of our Universe could possibly be made of normal matter, simply by measuring the hydrogen within it. Here’s how.
The Universe as we know it, at present, mostly adds up, but not in any sort of intuitive way. The 20th century brought with it the realization that the majority of what we know and experience in this physical Universe — atoms, light, and the known subatomic particles along with everything they combine to form — makes up only 5% of the total amount of stuff in the Universe. The remaining 95% is a mixture of things that are completely “dark” to us: dark matter, which gravitates, and dark energy, which drives the expanding Universe ever farther apart. Uncovering the nature of the dark Universe remains one of the most ambitious goals for 21st century science.
Our best evidence for the dark Universe comes from the combination of three different independent lines of observation:
- the cosmic microwave background,
- the large-scale clustering structure of the Universe,
- and the observed redshift-distance relation of objects, such as type Ia supernovae, at all cosmic distances.
They indicate, combined, that our Universe is only 5% normal matter, with 27% being dark matter and 68% being dark energy. While it’s easy to attempt to poke holes in this consensus cosmology picture, it’s been incredibly resilient.
Remarkably, there’s an easy and straightforward way — completely independently — to show that only ~5% of the Universe, total, can be made of normal matter: by looking at hydrogen and its rare, heavy isotope, deuterium. Here’s how measuring this “heavy hydrogen” reveals the existence of the dark Universe.
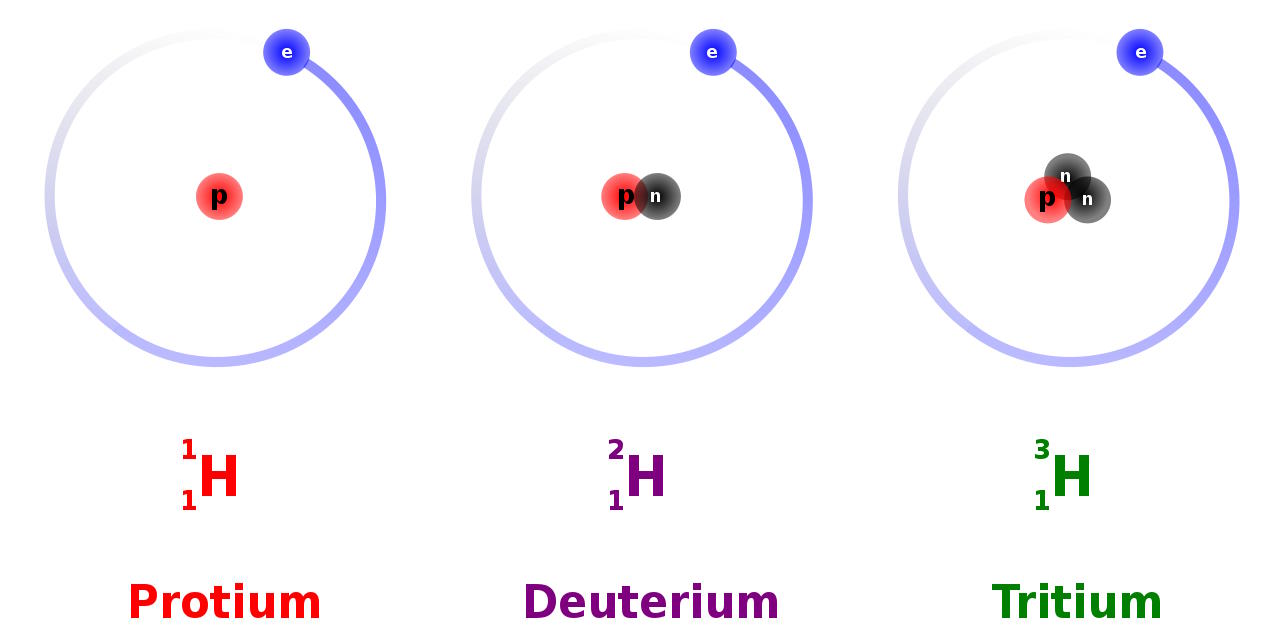
The key in understanding how measuring hydrogen tells us what’s in the Universe is to go through, step-by-step, the evolution of the young Universe shortly after the hot Big Bang. Once we determined that the Universe was:
- filled with galaxies,
- that were receding from one another,
- and that the more distant they were, the faster they appeared to recede,
it wasn’t long before we put together the picture of the expanding Universe. As the Universe expands, it not only gets less dense while gravitating and clumping together, it also cools, as the radiation within it gets stretched to longer and longer wavelengths.
If we run the clock representing the timeline of our Universe backward in our imaginations instead of forward, we’d determine that the Universe must have been smaller, denser, and hotter in the past. Extrapolate back far enough, and you’ll predict that the Universe began a finite amount of time ago in an event known as the hot Big Bang. If the Universe was hotter and denser in the past, but cooled, that means there was once a time where neutral atoms couldn’t form; that epoch is where the signal of the cosmic microwave background comes from. But even before that, there was a time when atomic nuclei couldn’t stably form, as the ultra-hot radiation background would blast any temporarily bound nuclei apart into their constituent protons and neutrons.
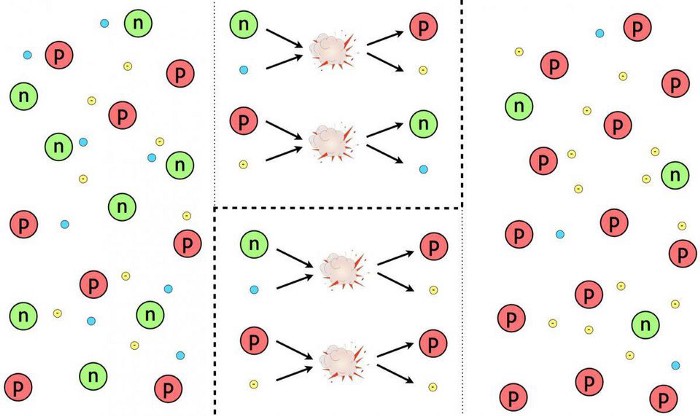
It’s by examining this part of the cosmic story in detail that we can understand a little more about the cosmic importance of hydrogen and its isotopes. If we were to go back to the very early stages of the hot Big Bang, to when the Universe was just a fraction-of-a-second old, we’d see a very different Universe from the one we recognize today. There were lots of free protons and neutrons, at temperatures and densities far in excess of what we find in the Sun’s core at present, along with a bath of photons and a number of particle-antiparticle pairs in great abundance. But there were no heavier nuclei, as the photons that were around at the time were so energetic that they’d immediately blast a heavier nucleus apart.
In order to stably form heavy atomic nuclei, we have to wait for the Universe to cool. As time goes on:
- electrons and positrons, the lightest charged particles, annihilate away, leaving only enough electrons to balance out the protons (and the electric charge) in the Universe,
- neutrinos stop interacting after previously and rapidly interconverting protons into neutrons and vice versa, leaving them to “free stream,” or travel without colliding with (and potentially transmuting) other particles,
- a fraction of the remnant free neutrons, with a half-life of around 10 minutes, radioactively decay into protons, electrons, and anti-electron neutrinos,
- and finally, only after 3–4 minutes, the Universe cools enough to successfully take the first step in forming heavy elements: fusing a proton and a neutron into deuterium, the first heavy isotope of hydrogen.

When the Universe was a fraction-of-a-second old, there was about a 50/50 balance between protons and neutrons. By the time three-and-change minutes have passed, that balance has shifted to about 87.5/12.5 in favor of protons, and it’s at only at this point that the Universe cools enough to pass this “deuterium bottleneck.” At last, nuclear fusion of the lightest elements can finally proceed unabated.
But by the time that 3-to-4 minutes have passed since the hot Big Bang, the Universe is a lot cooler and less dense than it once was. Temperatures are still high enough to initiate nuclear fusion, but the density — due to the expansion of the Universe — is only about 0.0000001% of what it is in the Sun’s center. As a result, most of the neutrons that still remain wind up combining with protons to form helium-4, with a small amount of
- helium-3,
- deuterium,
- tritium (which decays to helium-3),
- and isotopes of lithium
- and beryllium (which eventually decay to lithium)
also forming and remaining from this epoch.
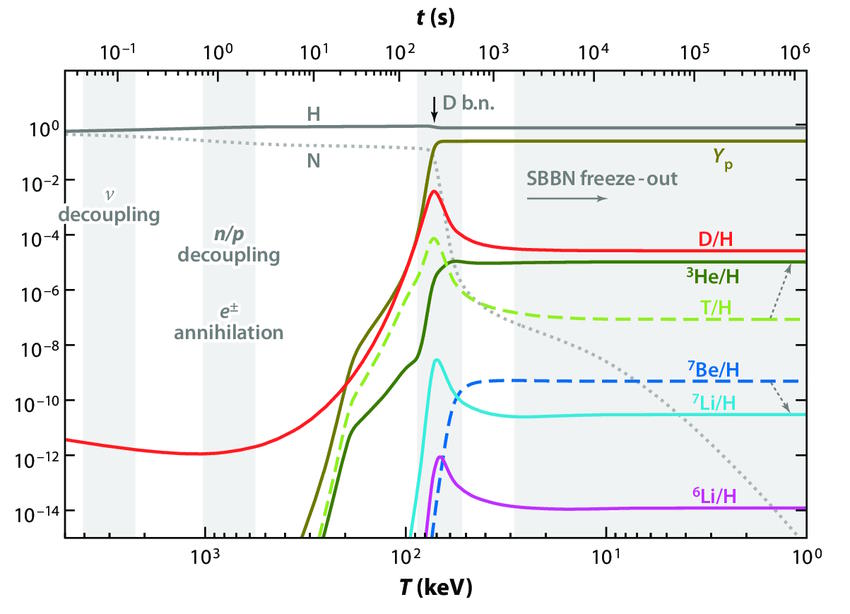
What’s remarkable about these predictions is how little wiggle-room the Universe gives them for what the overall outcome can be. Given the Standard Model of particle physics, and how nuclear processes are known to work, there should be a particular ratio of the light elements that survive today that depends on one property of the Universe alone: the ratio of baryons (protons and neutrons combined) to photons. (We sometimes label this parameter with the Greek letter η, the baryon-to-photon ratio.)
This is such a powerful technique because the number density of photons from the Big Bang is so precisely known: it’s been measured to be 411 photons per cubic centimeter at present, and so long as we understand how the Universe has expanded over its lifetime, we can infer just how many photons were present in a given volume of space at any point in the cosmic past.
Even completely independent of the imperfections in the radiation from the cosmic microwave background (i.e., the peaks-and-valleys in the temperature fluctuations on different scales), measuring the relative abundances of the light elements will tell us what the total amount of “normal matter” present in the Universe must be. In particular, we can see that measuring deuterium’s abundance, particularly if we can measure it precisely, will reveal to us the baryon-to-photon ratio of the Universe.

One problem, of course, is that these calculations we’re performing yield predictions for what the Universe was born with, but that’s not necessarily the same as the Universe as we see it today. By the time we get to the stars and galaxies we can observe, the normal matter that exists has gone through processing:
- stars have formed,
- lived,
- burned through their nuclear fuel,
- transmuted light elements into heavy ones,
- and have recycled those processed elements back into the interstellar medium.
When we look at stars today, they don’t exhibit these predicted ratios, but significantly altered ones. In addition to these light elements, there are also heavy ones showing up ubiquitously, like oxygen, carbon, and iron, among others.
In a Universe without pristine stars, how could you possibly try and reconstruct how much deuterium was present immediately following the Big Bang?
One method you might consider is to measure the ratios of elements in a variety of stellar populations. If you measure, say, the oxygen-to-hydrogen or iron-to-hydrogen ratios, and also measure the deuterium-to-hydrogen ratio, you could graph them together, and use that information to extrapolate backward: to a zero oxygen or iron abundance. This is a pretty solid method, and gives us an estimate for how much deuterium would be present at a time before heavy elements, like oxygen or iron, had formed.

But ideally, you’d want to probe the deuterium abundance directly: in as close to a pristine environment as possible. If you’ve already formed stars, you’ve probably both made and/or destroyed deuterium via nuclear processes, which throw your conclusions into doubt. Ideally, you’d want to find gas that was as close to pristine as possible, without the associated pollution of the stars themselves. You’d want to get high-precision measurements of clouds of gas — ideally very far away, corresponding to very far back in time — with no stars in them at all.
This seems like an impossibility, until you realize that clouds of gas can absorb light, imprinting the unique signatures of whatever elements they possess inside of them onto that background light. The brightest, most luminous light sources from the distant universe are quasars: supermassive black holes that are actively feeding in galaxies at great distances. Everywhere there’s an intervening cloud of gas, a portion of that quasar light gets absorbed, as whatever atoms, molecules, or ions that are present will absorb that light at those explicit quantum frequencies particular to whatever particles are present at whatever redshift they’re located at.
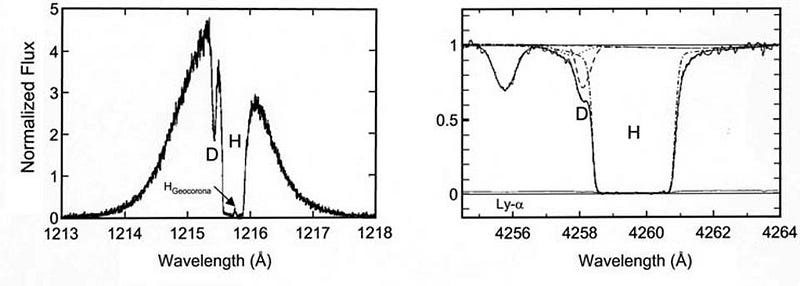
You might think that deuterium, being an isotope of hydrogen, would be indistinguishable from hydrogen itself. But when it comes to the frequencies at which atoms emit or absorb light, they’re determined by the energy levels of the electrons in that atom, which depend on not just the charge of the atomic nucleus, but on the ratio of the electron mass to the mass of the nucleus itself. With an extra neutron in its nucleus, the deuterium absorption line overlaps with, but its peak is off-center from, the peak of the normal hydrogen.
By looking at the best quasar data we have in the Universe, and finding the closest-to-unpolluted molecular clouds that exist along their lines-of-sight, we can reconstruct the primordial deuterium abundance to extreme precision. The latest results tell us that the amount of deuterium in the Universe, by mass, was 0.00253% of the initial hydrogen abundance, with an uncertainty of only ±0.00004%.
This corresponds to a Universe that’s made up of about 4.9% normal matter: consistent to within ~0.1% of the value inferred from the imperfections in the cosmic microwave background radiation, but in an entirely independent way.

One question you might wonder, of course, is whether we’ve worked out the underlying nuclear physics correctly. After all, there’s a big difference between “We understand the laws of physics and how the equations work, and here’s what we predict,” and “We recreated the conditions that were present and demonstrated that the outcomes are in line with our theoretical predictions.” The first allows us to make a prediction — which we can then compare with our observations — but the second would experimentally confirm that our predictions are actually worth their weight in heavy isotopes.
The way we frequently approach problems like this is to identify which step in the process is the most uncertain, particularly if the uncertainty in that step is greater than the uncertainty in either:
- the observational data we have to compare our results with,
- or the desired precision of our end conclusion.
For the nuclear processes involved in both creating and burning deuterium, that’s where deuterium fuses with a proton to form helium-3, an uncommon, light, but stable isotope of the element helium.

In November of 2020, at an underground laboratory in Italy, a plasma physics experiment at the Laboratory for Underground Nuclear Astrophysics (LUNA) published a paper detailing what they saw when they went and recreated the high temperatures and densities that were present during the hot Big Bang. They observed the reactions between deuterium and protons directly in their experiment, which ran from 2017-2020. It took three years to measure enough different conditions to high-enough precisions to recreate the necessary temperature ranges, but when all was said and done, they had the best measurement of this particular reaction rate ever: with an uncertainty of just 1.6%.
Most importantly, though, it confirmed our expectations. Although the uncertainties had been larger, previously, the central value didn’t shift by very much at all, meaning that our estimates for how the deuterium abundance corresponds to and translates into an overall matter density was actually extremely good. The Universe, as best as we can tell, really is made of about 5% normal matter, and can’t be more than that. Even if we don’t know what dark matter’s nature is, this experiment shows us that practically none of it is allowed to be “normal matter that, for some reason, is just dark.”

This is a conclusion whose importance cannot be overstated. There’s an awful lot we don’t understand about our Universe today, including why we live in a Universe where so much of what exists lies beyond the reach of our observation. There are a lot of reasons to be skeptical of dark matter and dark energy, for instance: they’re tremendously counterintuitive. Just because the cosmic microwave background tells us they must be there, for example, doesn’t mean they necessarily exist. If that one line of evidence is flawed — either from the data or our analysis — we don’t want our conclusions to be at risk of being overturned.
That’s why we demand multiple, independent lines of evidence for a conclusion before we confidently accept it. The science of Big Bang Nucleosynthesis is one of those incredibly important cross-checks. It’s an independent test not only of the Big Bang model of the early Universe, but of our concordance cosmological model. It tells us, all on its own, what the total amount of normal matter in the Universe is. Since the other lines of evidence, like colliding galaxy clusters or the large-scale structure of the Universe, require far more matter than the deuterium (or heavy hydrogen) abundance tells us can exist, we can be much more confident that dark matter is real. From the latest observations and experiments, only 4.7-5.0% of the entire energy budget of the Universe can be composed of normal matter. The remaining 95%, whatever it is, must truly be dark.