Ask Ethan: Is our Universe truly matter-dominated?
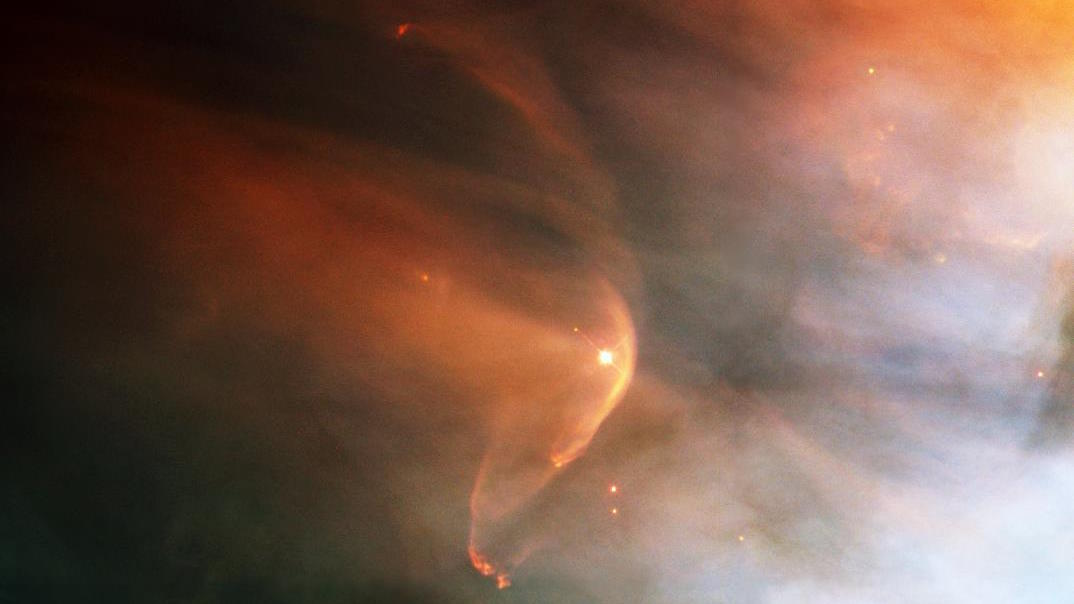
- We often state that our Universe is made of 4.9% normal matter, with virtually no antimatter, and that no one knows how this matter-antimatter asymmetry came to be.
- But how certain are we of this, truly? Could any of the distant stars, galaxies, or galaxy clusters actually be made of antimatter, and we simply don’t know it?
- Surprisingly, we have tremendously strong constraints on what the Universe is like, and we do very much know that we have a matter-antimatter asymmetric Universe. Here’s how.
Here in our own backyard, matter is common, while antimatter is rare. In fact, except for high-energy reactions that produce equal amounts of matter and antimatter — things like electron-positron pairs, for example — there’s absolutely no antimatter found anywhere that we look. All of the planets, stars, gas, dust, and more within our Milky Way are made of matter and not antimatter. All of the galaxies we look out at beyond our own are made of matter and not antimatter. Galaxy clusters and the large-scale cosmic web point to everything being made out of matter and not antimatter. Somehow, all of the normal stuff, the stuff of the Standard Model, is all “matter” in our Universe, with practically no antimatter at all.
Most of the time, we ask the big question of baryogenesis: how did the Universe come to be made of matter and not antimatter? But before we even get there, are we truly, absolutely certain that the Universe is made of matter, and that there isn’t some large collection of antimatter out there? That’s what Tim Thompson wants to know, asking:
“How do we know that it’s predominantly one over the other? Can we tell, from a distance, if a system is matter or antimatter? For example, for a galaxy millions of light years away, which we only observe via photons emitted, what is it that tells us its matter vs antimatter?”
It’s a great question. And thankfully, astronomy and astrophysics holds the answer.

Whenever matter and antimatter meet in the Universe, they annihilate, and matter-antimatter annihilation produces a very specific signal. When a particle of matter collides with its antimatter counterpart, it typically results in the production of two photons (in the center-of-momentum frame of reference of the collision) with equal energies and opposite momenta. An electron annihilating with a positron, for instance, produces two photons of precisely 511,000 electron-Volts of energy apiece: the energy equivalent of the mass of the particles that annihilated, via Einstein’s E = mc².
We can see these annihilation signals throughout space wherever they occur, enabling us to identify where matter and antimatter meet. If there were:
- planets,
- stars,
- galaxies,
- clusters of galaxies,
- or even intergalactic regions of space,
where some were matter and some were antimatter, we’d see evidence of those high-energy photons from annihilation at the interface. The fact that we do see those photons, but so rarely and only in specific locations (mostly consistent with emissions from pulsars and active black holes), allows us to place tremendous constraints on what fraction of the Universe, on a variety of scales, can be made of antimatter.
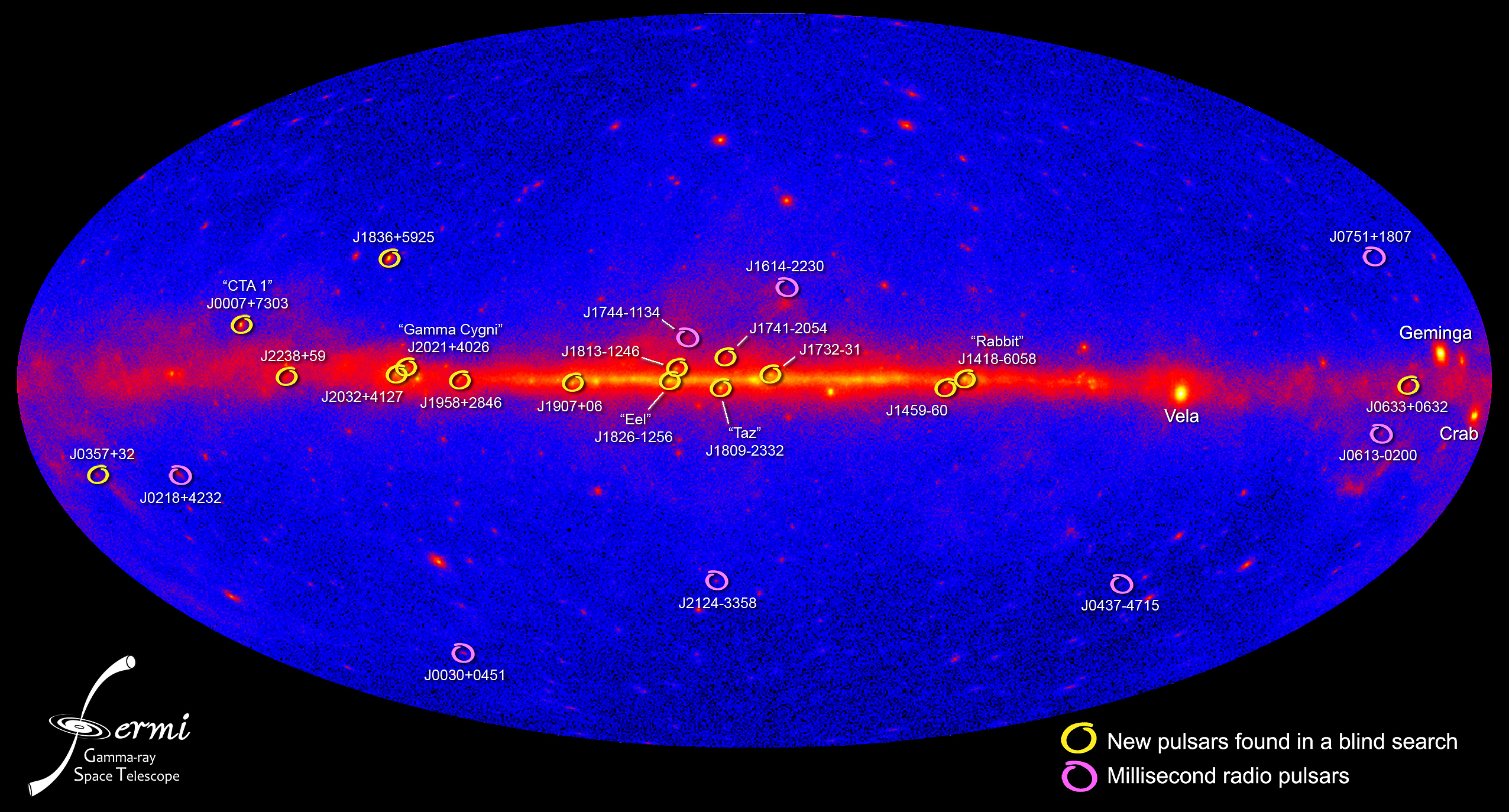
Within a galaxy, you have to recognize that stars aren’t just isolated objects, but rather have extended structures around them: planets and moons, zodiacal dust in a plane, a Kuiper-like belt and a scattered disk, and an Oort-like cloud around them, spanning around a light-year in any direction. A few times in every million years — and remember, we’re already living in a Universe that’s 13.8 billion years old (or, to make things clearer, 13,800 million years old) — another star/star system will pass within one light-year or less of any given star. That means, over a star’s lifetime, it should experience thousands of interactions with another star/star system within our galaxy.
If there were any antimatter stars, complete with antimatter planets, antimatter moons, and antimatter bodies in their disk and the surrounding cloud, there would be a tremendous release of energy whenever the antimatter from that system interacted with the matter from the remaining stars in our galaxy. The fact that we don’t routinely see high-energy emissions, such as gamma-ray bursts, coming from within our galaxy tells us very strongly that there are no antimatter stars within our galaxy. The fact that we don’t see it in nearby galaxies severely constrains the amount of antimatter that could be present within them.

We can scale this problem up to larger cosmic scales as well. Within galaxy groups and galaxy clusters, there are plenty of observations of galaxies moving through these clusters, some of which move through them at breakneck speed. We find plenty of evidence for stars and gas within the intracluster medium (the space between the galaxies within the cluster), and this gas interacts with the galaxies that move through that space. We see the effects of gas stripping, tidal disruption, and star formation in and around these galaxies. But, at the same time, there’s no evidence of matter-antimatter annihilation.
In other words, when we look at a galaxy group or a galaxy cluster, if any of the galaxies within them were made of antimatter, we would see the effects of matter-antimatter annihilation where these antimatter galaxies interact with the remainder of the group or cluster. The fact that we’ve observed thousands upon thousands of galaxy groups and clusters in the Universe, and never once have encountered a signal that would be consistent with this type of matter-antimatter annihilation, severely constrains how much antimatter could be out there.

And on the largest cosmic scales of all, we can look at three different sets of systems.
- We can look at groups of galaxies that are colliding and merging with one another.
- We can look at separate galaxy clusters that are undergoing the process of colliding.
- And we can even look at the large-scale cosmic web, where enormous structures — collections of galaxies — can gather in filaments exceeding a billion light-years in length.
In all of these systems, we find evidence for all the complex physics we expect to see if everything in the system is made of the same type of matter: either 100% matter or 100% antimatter.
We see gas heating up and emitting X-rays where collisions occur. We see the evidence of this material separating from dark matter, as the “normal” stuff experiences drag, heating, and the formation of new stars, but the dark matter simply passes right through itself and the normal stuff unimpeded. We see the emitted light rotating in its polarization (Faraday rotation), consistent with the presence of magnetic fields on galactic scales. And, again, we see an absolute lack of matter-antimatter annihilation, teaching us that there are no “matter” regions and “antimatter” regions coming into contact with one another.
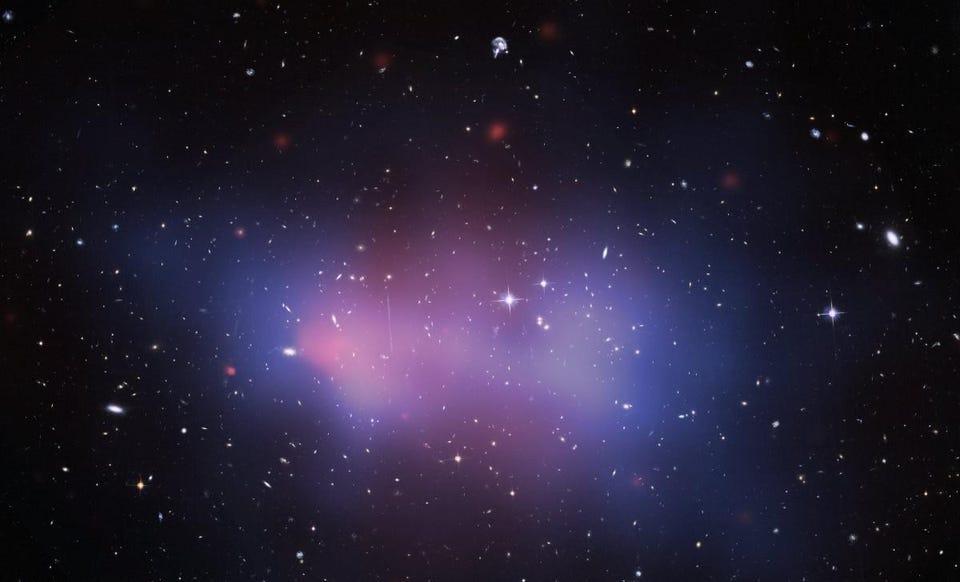
It’s also possible that if our Universe was born with a network of topological defects, including:
- 1-dimensional defects, such as cosmic strings,
- 2-dimensional defects, such as domain walls,
- or 3-dimensional defects, such as cosmic textures,
we could have a discontinuity: where matter dominates on one side of the defect and antimatter dominates on the other side of the defect.
Unfortunately for these scenarios, they’ve all been ruled out with extraordinary confidence due to the large-scale clustering data in the Universe, as well as by detailed analyses of the cosmic microwave background. There are a number of theoretical mechanisms that one could propose to create separated regions in space, where one region contains matter and one contains antimatter, but all of them have at least one of the following two things in common:
- They create a discontinuity in the clustering data of the Universe, one that would have appeared in galaxy surveys.
- They create an interface between the matter and antimatter regions, which would lead to lines, sheets, or broader regions where matter and antimatter would annihilate.
The fact that these features are observationally absent means we can strongly conclude that our Universe, for all intents and purposes, is 100% matter, and only a negligible amount of antimatter.
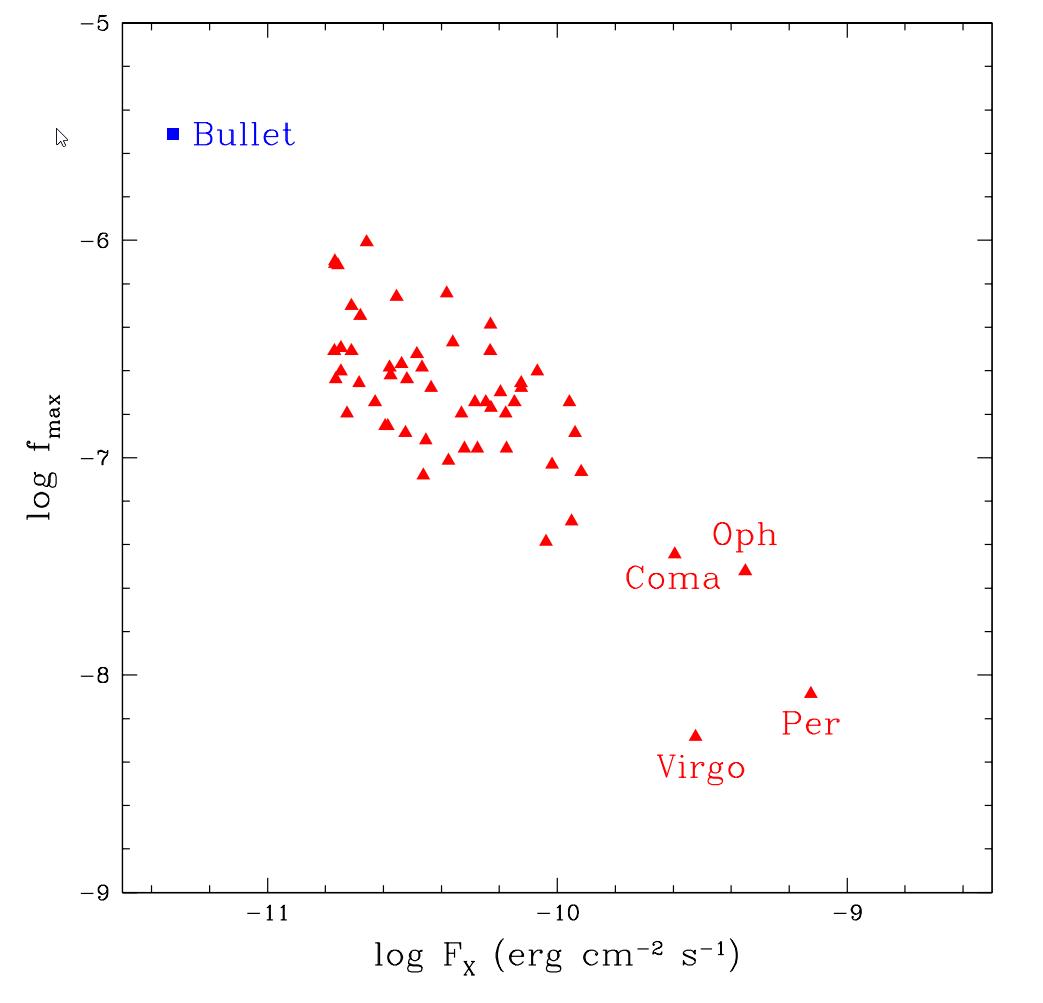
But let’s say you wanted a wholly independent line of evidence to look at to determine the matter abundance in the Universe. Would such a thing, independent of stars, galaxies, clusters of galaxies, and the gamma-ray sky to point to, actually exist?
Indeed it would: we have the abundance of the light elements, formed during the early stages (the first few minutes) of the hot Big Bang, that were created during the earliest stages of nucleosynthesis.
Since the energy of each light wave is defined by its wavelength, and the Universe expands over time, the wavelength of each photon gets stretched as time marches forward. If we extrapolate backward instead, however, we find that the wavelength of each photon was shorter — more compressed — in the past, meaning that the farther back we look in time, the hotter the Universe was back in those early stages. At some point, the Universe was so hot that neutral atoms were an impossibility to form, as there were not enough photons of sufficient energy to prevent electrons from stably binding to the atomic nuclei that were present. But if we like, we can go back even farther than that.

We can go all the way back to an epoch where the Universe was so hot that even atomic nuclei couldn’t bind together. Every time they would attempt to do so, a photon would blast the individual protons and neutrons apart, preventing them from building up to heavier elements. Only when the Universe has cooled below a certain critical threshold — which occurs about 3 to 4 minutes after the start of the hot Big Bang — can we then start forming atomic nuclei that are heavier than a single, simple proton.
Once that moment arrives, we can build up the lightest elements in the Universe according to the rules of nuclear physics. Remarkably, the ratio of the light elements and their isotopes that we get out, including:
- hydrogen (a single proton),
- deuterium (a proton plus a neutron),
- helium-3 (two protons plus a neutron),
- helium-4 (two protons and two neutrons), and
- lithium-7 (four protons and three neutrons),
depends on only one parameter: the ratio of photons to the total number of protons and neutrons combined. When we take observations, both from the most pristine clouds of gas we can find and also from the imprint in the cosmic microwave background, we get the same answer: there’s about 1 proton or neutron for every 1.6 billion photons in the Universe. Even in the very early stages of the hot Big Bang, there was more matter than antimatter.

On the one hand, this is a good thing. If there were equal amounts of matter and antimatter in the Universe, almost all of it would have annihilated away. At present, there would be less than one particle of either matter or antimatter per cubic kilometer in the Universe remaining.
As it stands, however, the Universe is much denser than that by about a factor of a billion, and practically all of what remains is matter, not antimatter. But the only way we know of to convert energy into mass or to convert mass into energy always has the same result: the number of matter particles minus the number of antimatter particles is always a constant.
Somehow, there has to be something else going on with the particles in the Universe — beyond what the Standard Model predicts — to create the Universe as we observe it to be today. If we approach the problem scientifically, that means extrapolating back to the earliest state of the hot Big Bang, where particles and antiparticles of all types could easily be created at the highest energies, and to see what it would take for the Universe to create a matter-antimatter asymmetry where there was none initially.
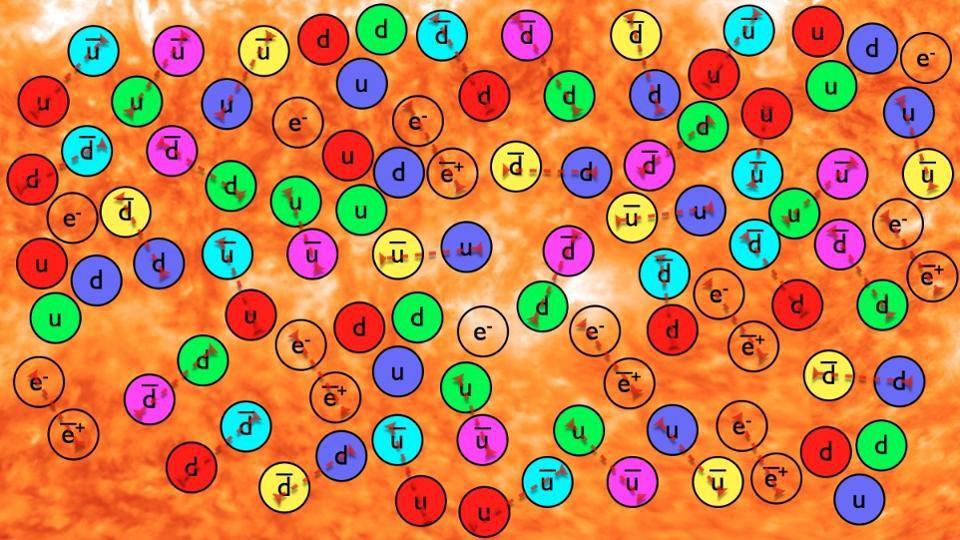
This is why we care so strongly about the problem of baryogenesis, or how there came to be more matter than antimatter in the Universe. Yes, there are some general things we can say about how to create one from an initially symmetric state, as was shown by Soviet physicist Andrei Sakharov, back in 1967. All you need to do is meet the following three criteria, known as the Sakharov conditions:
- The Universe must be out of thermal equilibrium.
- The Universe must contain examples of both C-symmetry and CP-symmetry violation.
- And the Universe must admit interactions that violate the conservation of baryon number.
While we don’t know the exact mechanism behind how the Universe came to have more matter than antimatter, we know that it was a necessary step in permitting our Universe, and the objects and creatures in it, to exist as they do. Numerous experiments from around the world are constantly probing matter and antimatter at subatomic scales, searching for any hints of baryon-number violation and for additional C-symmetry and CP-symmetry violating interactions.
However, a Universe that doesn’t have more matter than antimatter in it is thoroughly ruled out by observations. We might not have found the “tree of life” that allowed for our very existence, but thanks to the physics we know so far, we can be confident we’re at least looking in the right forest.
Send in your Ask Ethan questions to startswithabang at gmail dot com!