How the surprising muon revolutionized particle physics
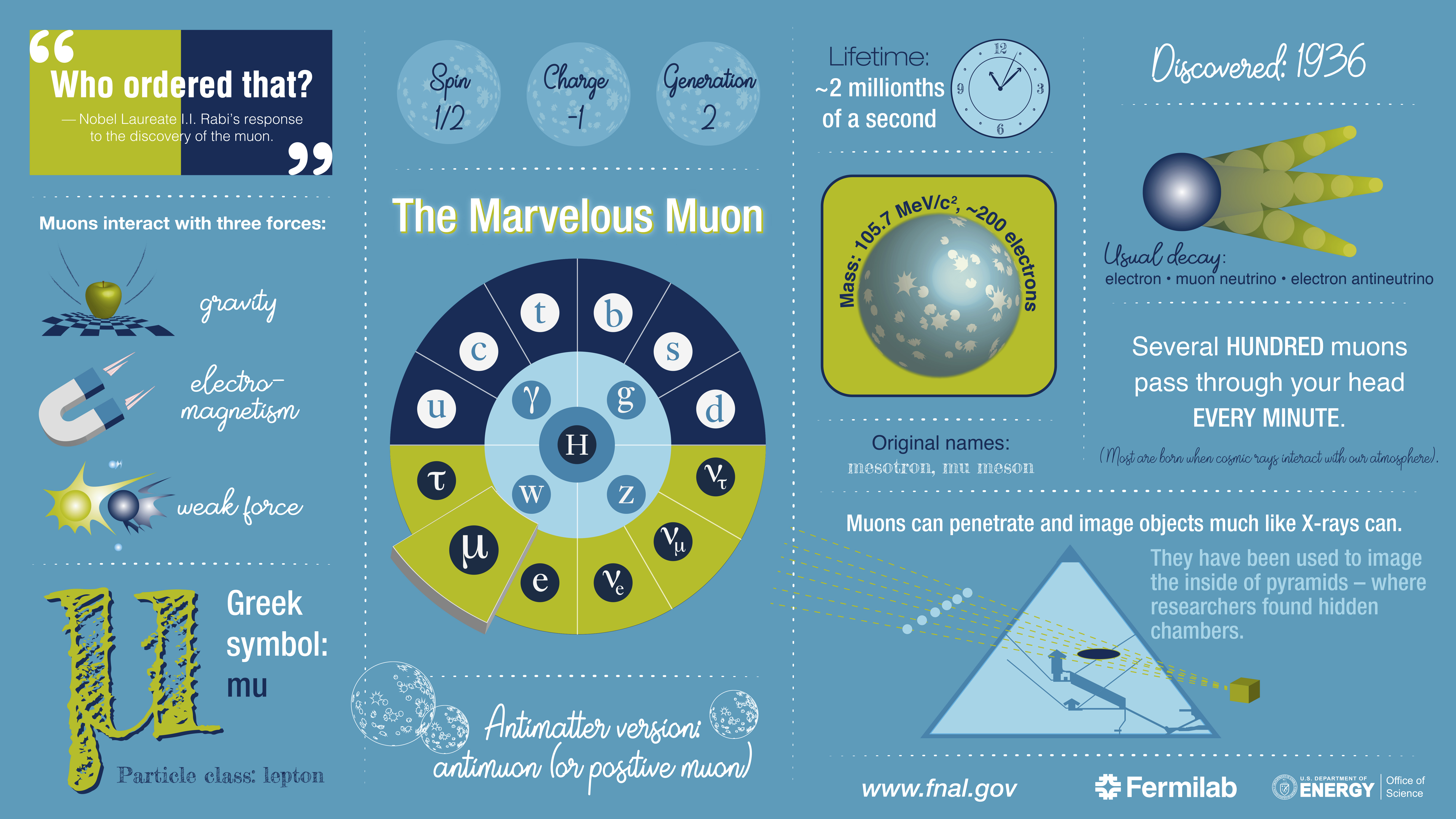
- Back in the 1930s, there were only a few particles that were needed to explain all of what was known in existence: the proton, neutron, electron, and photon.
- Although, according to some of the novel theories of the day, new particles like the neutrino and the positron were expected, what actually showed up was a complete weirdo: the unstable muon.
- This particle, which lived for just microseconds and was similar to the electron but hundreds of times heavier, turned out to be the key to unlocking the secrets of the Standard Model. Here’s how it revolutionized particle physics.
Back in the early 1930s, there were only a few known fundamental particles that made up the Universe. If you divided up the matter and radiation we observed and interacted with into the smallest possible components we could break them up into at the time, there were only the positively charged atomic nuclei (including the proton), the electrons that orbited them, and the photon. This accounted for the known elements, but there were a few anomalies that didn’t quite line up.
Heavier elements also had more charge, but argon and potassium were an exception: argon only had a charge of +18 units, but a mass of ~40 atomic mass units, while potassium had a charge of +19 units, but a mass of ~39 units. The 1932 discovery of the neutron took care of that one, teaching us that the periodic table should be sorted by the number of protons in the atomic nucleus. Certain types of radioactive decay — beta decays — appeared to not conserve energy and momentum, leading to Pauli’s 1930 hypothesizing of the neutrino, which wouldn’t be discovered for another 26 years. And the Dirac equation predicted negative energy states, which corresponded to antimatter counterparts for particles like the electron: the positron.
Still, nothing could have prepared physicists for the discovery of the muon: an unstable particle with the same charge, but hundreds of times the mass, of the electron. Here’s how this surprise really did turn physics on its head.

The story starts way back in 1912, when adventuresome physicist and hot air balloon aficionado Victor Hess had the brilliant idea to take a particle detector with him high into the stratosphere on one of his hot air balloon flights. You might wonder what the motivation would be for this, and it came from an unlikely source: the electroscope (above). An electroscope is just two thin pieces of conducting, metal foil, connected to a conductor and sealed inside an airless vacuum. If you charge up the electroscope, either positively or negatively, the like-charged leaves of foil will repel each other, while if you ground it, it becomes neutral, and the foil leaves inside of it will go back to the uncharged position.
But here was the strange thing: if you left the electroscope alone, even in a fairly perfect vacuum, it still discharged over time. No matter how good you made your vacuum — even if you placed lead shielding around the vacuum apparatus — the electroscope still discharged. Moreover, if you performed this experiment at higher and higher altitudes, you’d find that the electroscope would discharge (and the foil leaves would fall) more quickly. This was where Hess got his big idea, imagining that high-energy radiation, with both high penetrating power and of extraterrestrial origin, was the culprit.
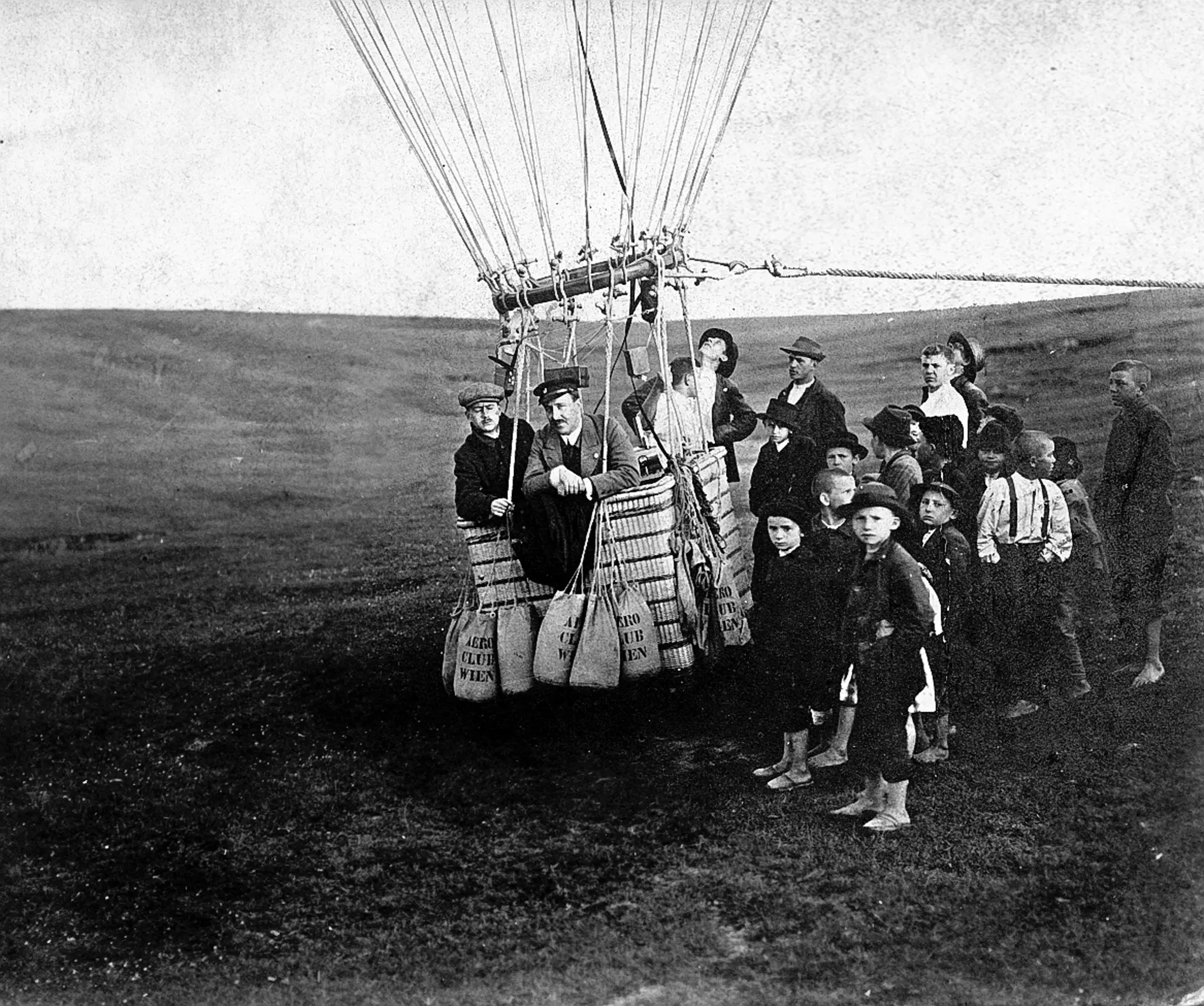
The idea was as follows: if there are charged cosmic particles zipping through Earth’s atmosphere, they could help neutralize any charge placed on the electroscope over time, as the oppositely-charged particles would be attracted to the electrode and the like-charged particles would be repelled by it. Hess imagined that there was a very real “zoo” of particles zipping around through space, and that the closer he got to the edge of Earth’s atmosphere (i.e., the higher altitudes he went to), the more likely it was that he’d be able to observe these particles directly.
Hess constructed a detection chamber that contained a magnetic field, so that any charged particles would curve and deflect in its presence. Based on the direction and curvature of any particle tracks that appeared in the detector, he could reconstruct the velocity of the particle as it moved through the detector, as well as what the particle’s charge-to-mass ratio was. Hess’s earliest efforts immediately paid off, as he began discovering particles in great abundance, founding the science of cosmic ray astrophysics in the process.
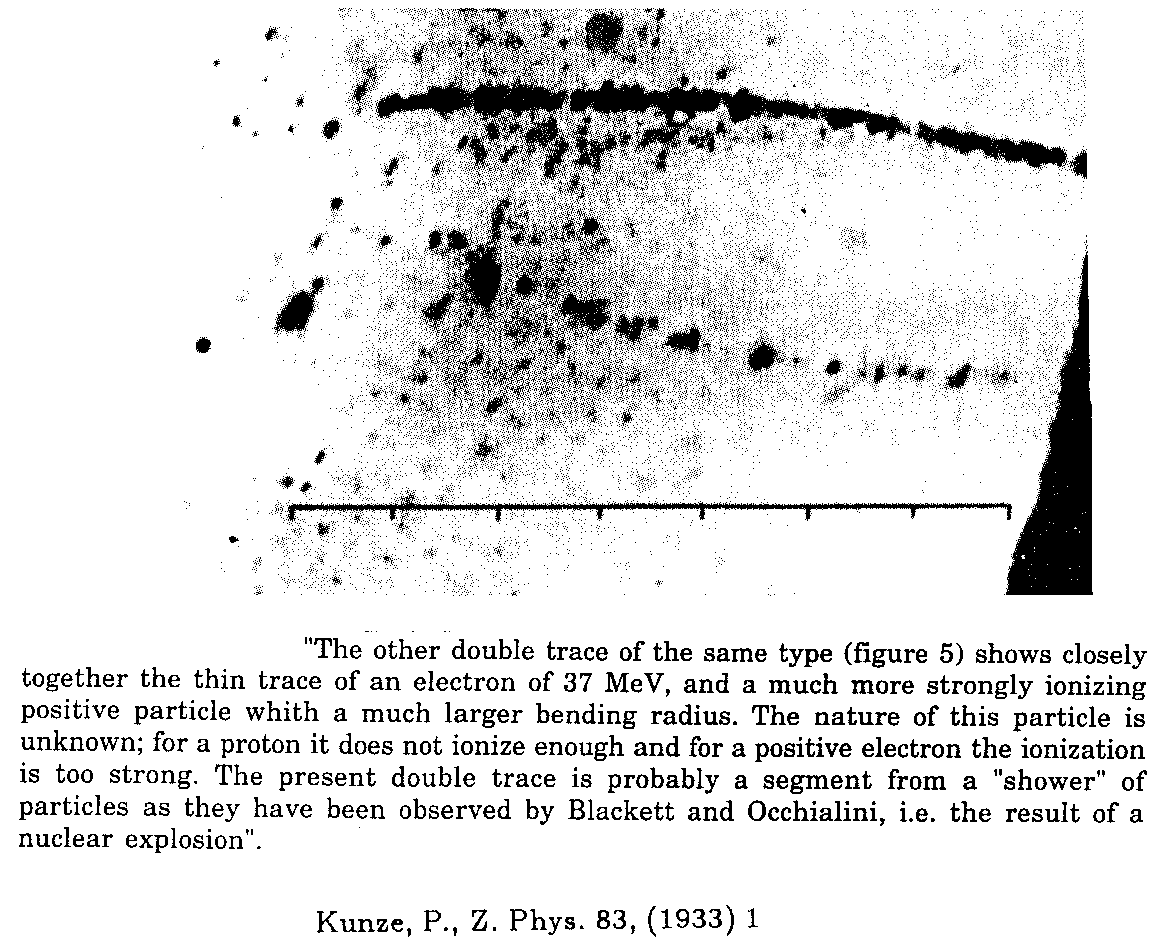
Many protons and electrons were seen in these early cosmic rays, and later on, the first antimatter particles (in the form of Dirac’s predicted positrons) were discovered this way as well. But the big surprise came in 1933, when Paul Kunze was working with cosmic rays and found a particle that didn’t quite fit any of the known species. The observed particle had the same charge as an electron, but was simultaneously far too heavy to be an electron while also being far too light to be an antiproton. It was as though there was some new type of charged particle, of an intermediate mass between the other known particles, that suddenly announced, “Hey, surprise, I exist!”
The higher in altitude we went, ever greater numbers of cosmic rays were observed. At the highest altitudes, the overwhelming majority of cosmic rays were neutrons and electrons and protons, while only a small fraction of them were muons. However, as detectors got more and more sensitive, they started to be able to detect these cosmic rays at lower altitudes, even close to sea level.
Today, for about $100 and with off-the-shelf materials, you can build your own cloud chamber and detect cosmic ray muons — the most abundant cosmic ray particle at sea level — at home.
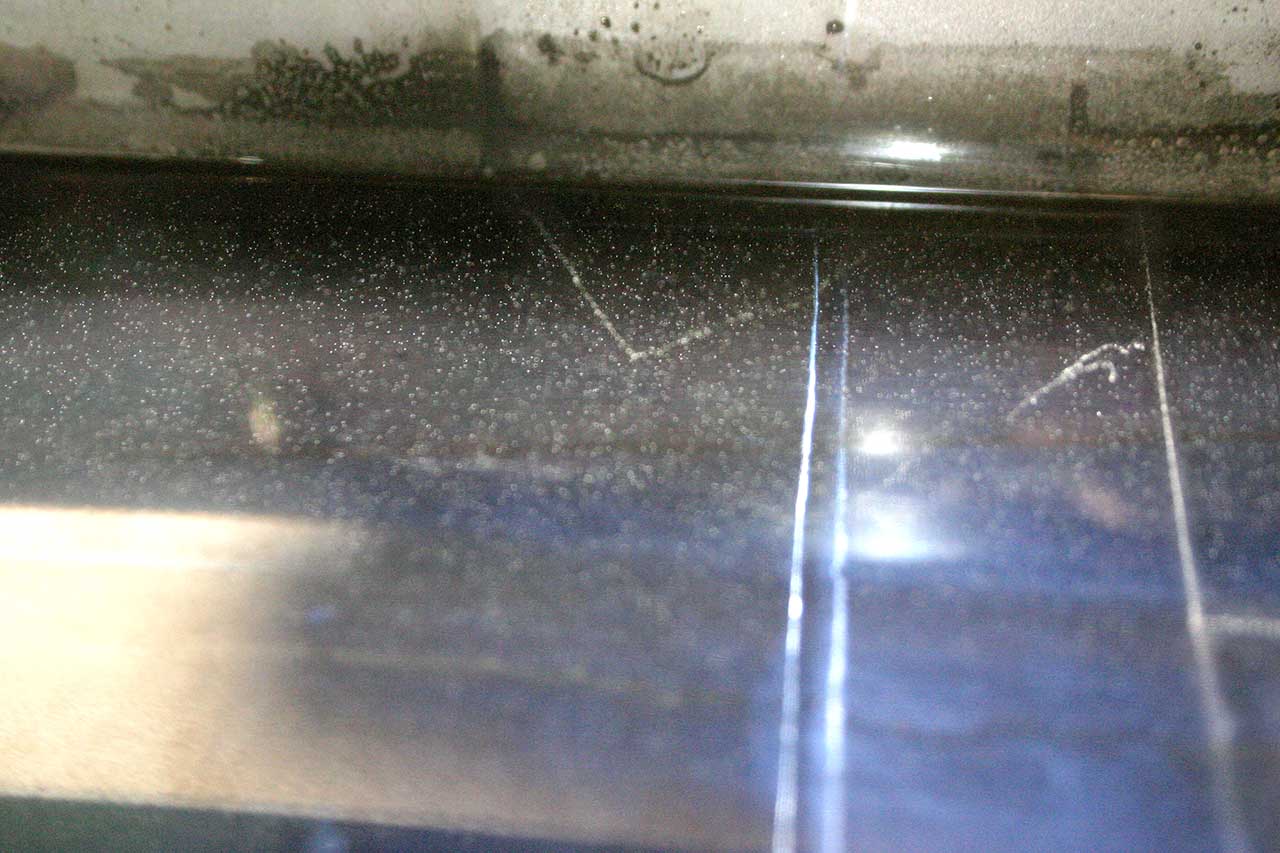
Over the next few years, scientists worked hard to detect these muons not merely from high-altitude experiments, but to observe them in a terrestrial laboratory. In theory, muons were being produced by what we call cosmic ray showers: where particles from space hit the upper atmosphere. When this occurs, interactions from the fast-moving cosmic particles that strike the stationary atmospheric particles produce lots of new particles-and-antiparticles, with the most common product being a charged, short-lived, unstable particle known as a pion.
The charged pions live only for nanoseconds, with negatively charged pions decaying into muons and positively charged pions decaying into anti-muons, along with other decay products. These muons and anti-muons are also short-lived, but much longer-lived than the pion. With a mean lifetime of 2.2 microseconds, they’re the longest-lived unstable particle except for the neutron, which has a mean lifetime of around 15 minutes! In theory, not only should the cosmic ray showers occurring in the upper atmosphere produce them, but any collision of particles that had enough energy to produce pions should also yield muons that could then be studied in a lab.
The muons, in our detectors, look just like electrons do, except they have 206 times the electron’s mass.
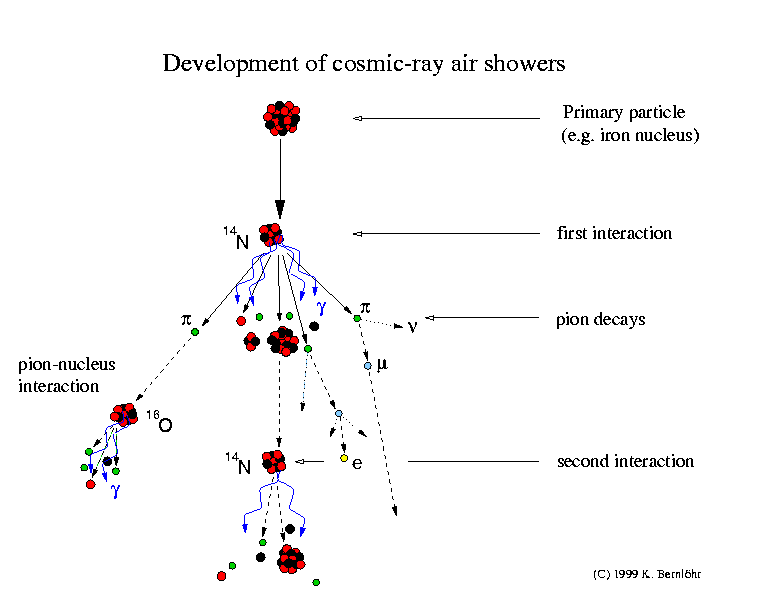
Once the muon had been observed, progress would occur relatively quickly in characterizing its properties and exploring its behavior. In 1936, Carl Anderson and Seth Neddermeyer were able to distinctly identify populations of both negatively and positively charged muons from cosmic rays, an indication that there were muons and anti-muons, just as there were electrons and anti-electrons (positrons) found in nature. That same year, Anderson and Victor Hess were jointly awarded the Nobel Prize in Physics for their early, pioneering work. The next year, 1937, saw the scientist team of J.C. Street and E.C. Stevenson independently confirm the discovery of muons and anti-muons in a cloud chamber. Muons were not only real, but relatively common.
In fact, if you hold out your hand and point your palm so that it faces up, toward the sky, approximately one muon (or anti-muon) will pass through your hand with each second that goes by. At sea level, 90% of all the cosmic ray particles reaching Earth’s surface are muons, with neutrons and electrons making up most of the rest. Before we had even discovered mesons, which are composite quark-antiquark combinations, exotic, heavy, unstable baryons (which are combinations of three quarks, like protons and neutrons), or the quarks that underlie matter, we had discovered the muon: the heavy, unstable cousin of the electron.
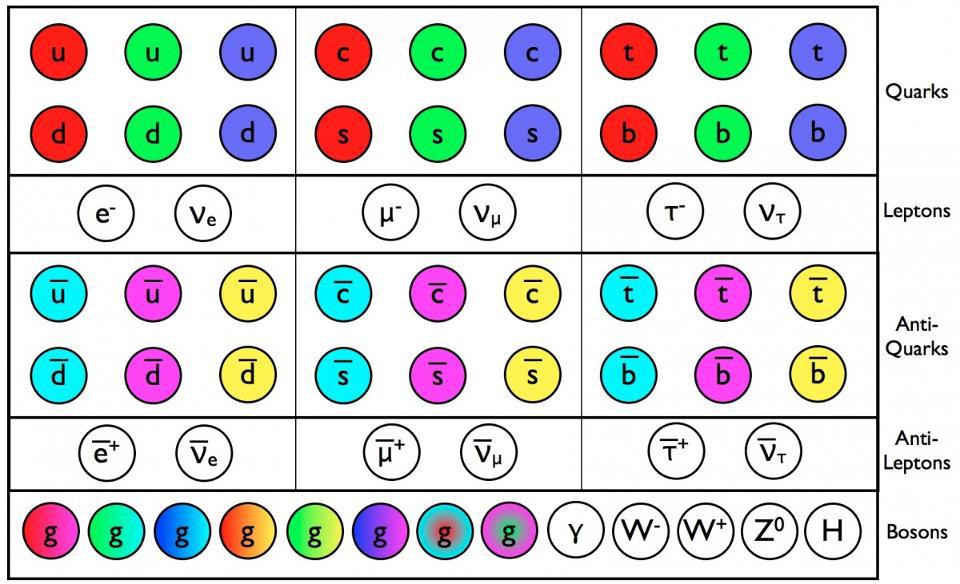
As soon as the physicist I. I. Rabi, who himself would win the Nobel Prize for the discovery of nuclear magnetic resonance (today used ubiquitously in MRI technology), learned about the muon, he famously quipped, “Who ordered that?” With so few particles known at the time, adding this strange cousin of the electron — heavy, unstable, short-lived, and seemingly unnecessary to explain the matter that made up our commonly experienced Universe — seemed like a phenomenon of nature that defied explanation.
We were decades away from uncovering the nature of matter and the structure of the Standard Model, but the muon was our very first clue that there were not only more particles out there waiting to be discovered, but that particles came in multiple generations. The first-generation of particles are the stable ones, consisting of the up and down quarks, the electron and the electron neutrino, and their antimatter counterparts. Today, we know of two more generations: the second-generation, which has charm and strange quarks with muons and muon neutrinos, and the third-generation, which has top and bottom quarks with tau and tau neutrino particles, plus their analogous antimatter counterparts.
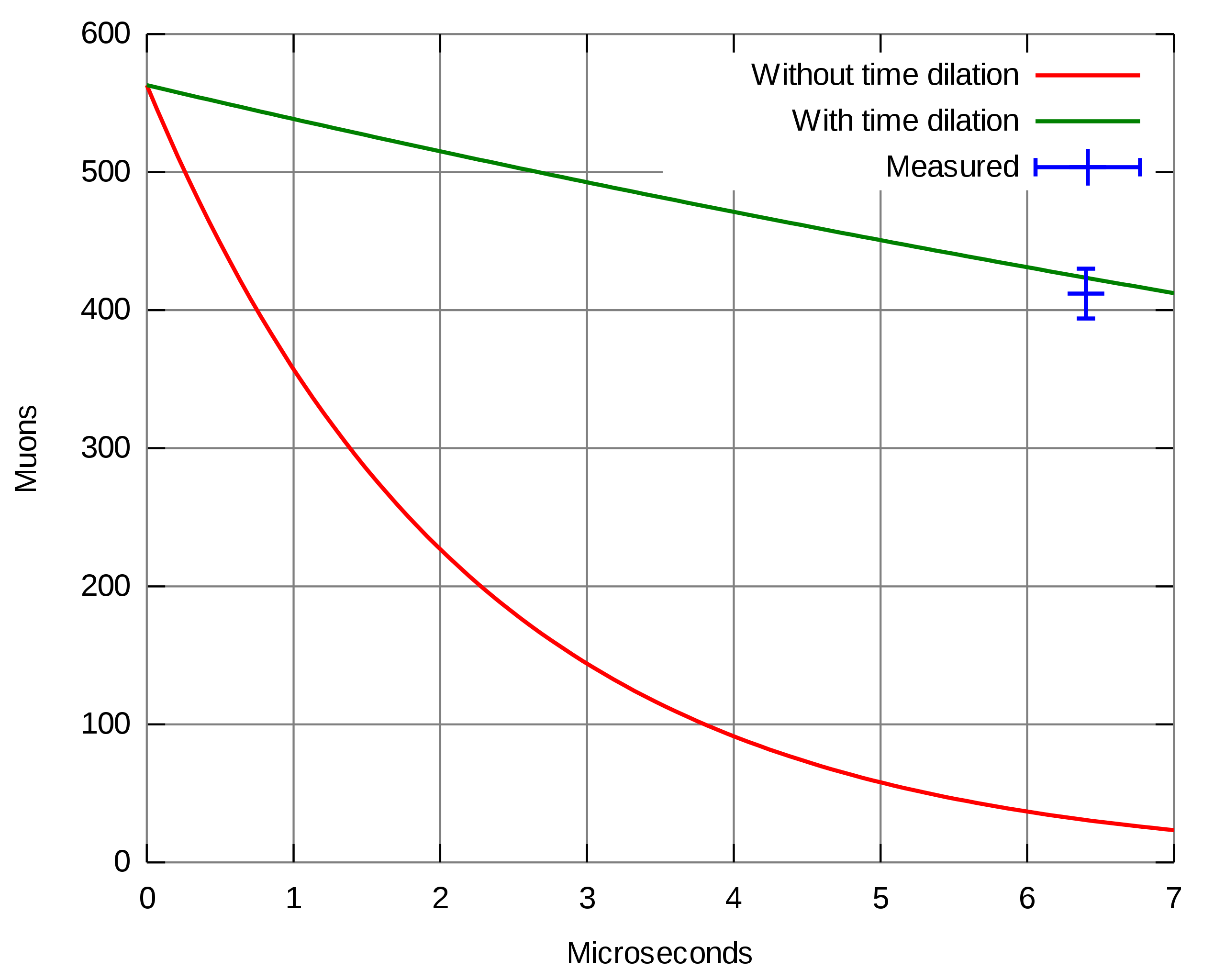
The muon, however, didn’t merely foreshadow all of these new discoveries, but it also yielded an exciting and counterintuitive demonstration of Einstein’s relativity. The muons that get created from cosmic ray collisions, on average, originate at an altitude of 100 kilometers. However, the mean lifetime of a muon is only 2.2 microseconds. If a muon moved extremely close to the speed of light at 300,000 km/s, you can do a little math, multiplying that speed by the muon’s lifetime, to find that they should travel about 660 meters before decaying.
But muons arrive at Earth’s surface, journeying in excess of 100 kilometers from when they were created, and still without decaying!
How is this possible?
Without relativity, it wouldn’t be. But relativity brings along the phenomenon of time dilation, enabling particles that move close to the speed of light to experience time passing more slowly than they do for observers at rest. Without time dilation, we would never have discovered these cosmic muons, and we wouldn’t be able to see them in our terrestrial cloud chambers, not unless we created them from particle accelerators. Einstein, despite not knowing it, helped us discover this fundamentally new form of matter.
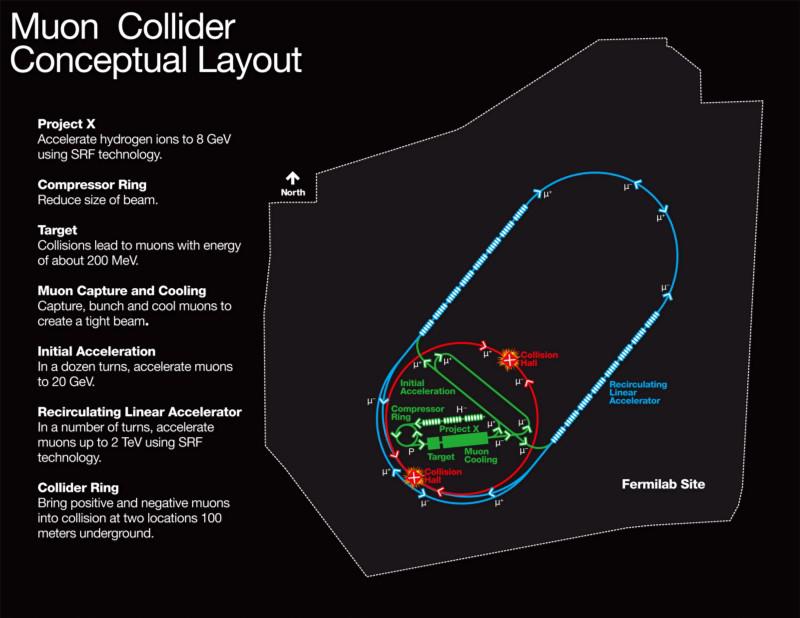
Looking ahead, being able to control and manipulate these muons just might lead to advances in experimental particle physics that no other type of collider can match. When you build a particle accelerator, there are only three factors that determine how energetic your collisions are:
- how big your ring is, with larger circumference rings achieving higher energies,
- how strong your magnetic fields that bend your charged particles are, with stronger magnets leading to higher energies,
- and the charge-to-mass ratio of your particle, with low masses leading to synchrotron radiation and a limiting energy, and high masses not having that problem.
That third factor is why we use protons instead of electrons in accelerators like the Large Hadron Collider at CERN, but there’s a drawback: protons are composite particles, and only a tiny fraction of their total energy winds up in the single quark or gluon that takes part in the high-energy collision that we wind up studying. But the muon doesn’t suffer from that drawback; it’s an elementary, fundamental particle, rather than a composite one. Additionally, muons aren’t limited by synchrotron radiation like electrons are, due to their much heavier masses. If we can master muon accelerators — i.e., create and confine muons to accelerate them to sufficiently high energies before they decay — we just might unlock the next frontier in experimental particle physics.
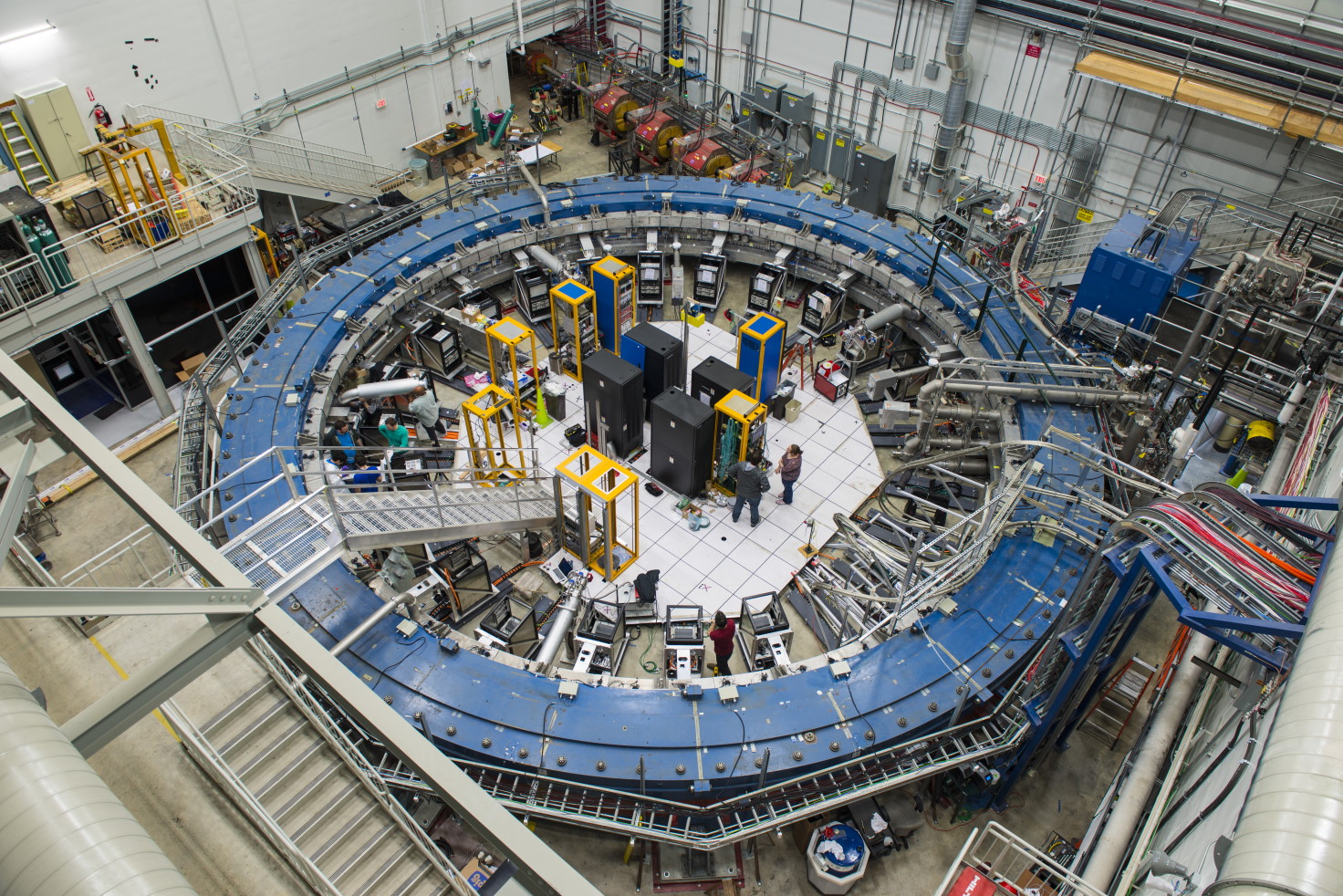
Today, we can look back on the discovery of the muon as quaint, with our hot air balloons and primitive detectors revealing these uniquely bent particle tracks. But the muon itself continues to provide a legacy of scientific discoveries. From its power in illustrating the effects of time dilation on a particle’s observed lifetime to its potential to lead to a fundamentally new, superior type of particle accelerator, the muon is a whole lot more than just background noise in some of our most sensitive, underground experiments searching for the rarest particle interactions of all. Even today, the experiment to measure the muon’s magnetic dipole moment could be the key that takes us, at last, into understanding physics beyond the Standard Model, and could reveal the possible existence of a fifth fundamental force of nature.
Still, when it unexpectedly announced its existence in the 1930s, it was truly a surprise. For all of history before then, no one had imagined that nature would make multiple copies of the fundamental particles that underpinned our reality, and that those particles would all be unstable against decay. The muon just happens to be the first, lightest, and longest-lived of all of those particles. When you think of the muon, remember it as the first “generation 2” particle ever discovered, and the first clue we ever received from nature as to the true nature of the Standard Model.