Ask Ethan: Do evolution and natural selection occur cosmically?
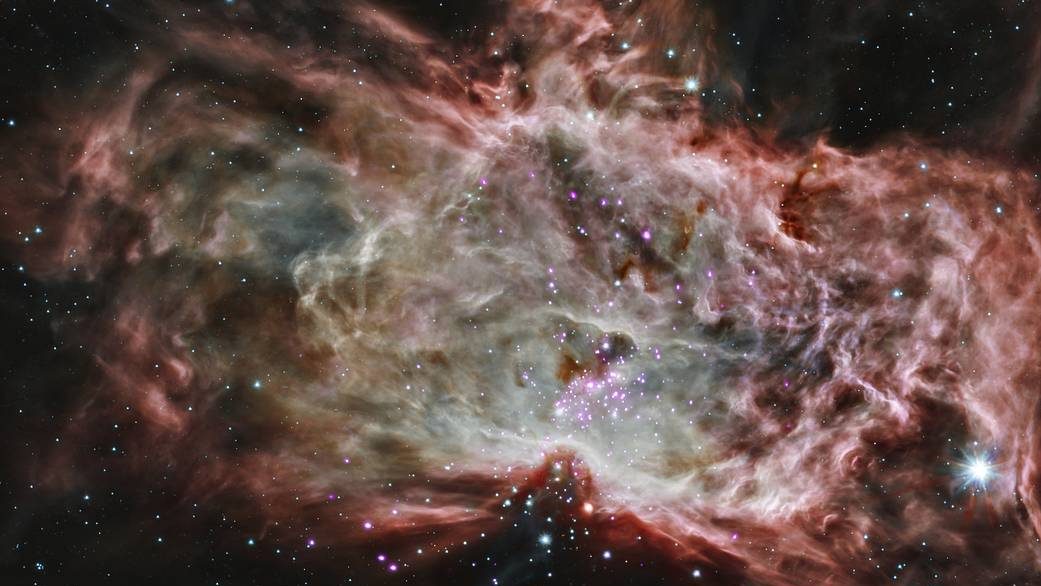
- In the world of biology, evolution is one of the most important ideas behind how organisms change over time, and natural selection is perhaps the most important mechanism by which evolution takes place.
- In the cosmos, evolution occurs as well, as we have gone from a hot, dense, uniform, and rapidly expanding Universe to one that contains stars, galaxies, a vast cosmic web, and even human beings in it.
- Does cosmic evolution obey similar principles to biological evolution? And if so, what’s the driving mechanism behind the evolution of our Universe? Science holds the profound answer.
Here on Earth, all living organisms obey certain rules and laws, and are subject to the phenomenon of evolution, including (and often, primarily) through the process of natural selection. Organisms, in terms of:
- the functions they can perform,
- the structures they possess,
- and the underlying genetic sequences that encode them,
all of which largely determine their biology, all change over time, or evolve. Some organisms, or even entire groups of organisms, will go extinct when resources run scarce or competitors arise, while others will survive, giving rise to future organisms whose lineages will persist. The survivors are selected for, naturally, while those who go extinct are selected against, naturally.
Although the mechanism of natural selection was only uncovered in the 1800s, with the work of Alfred Russell Wallace and Charles Darwin, there are certainly analogous processes of evolution and — from a certain point of view — natural selection that occur on cosmic scales as well. How far, though, can we responsibly take this analogy? That’s at the heart of what Pat Connolly wants to know, who writes in to ask:
“Does evolution and natural selection occur in the cosmos, and if so what physics is involved and how has it made the cosmos “better” over time?”
I want to stay away from a value judgment like “better” when it comes to talking about the physical entities in the Universe, but yes, cosmic evolution does occur, and certain physical structures — again, in a sense — are selected for, while others are selected against. Here’s how.
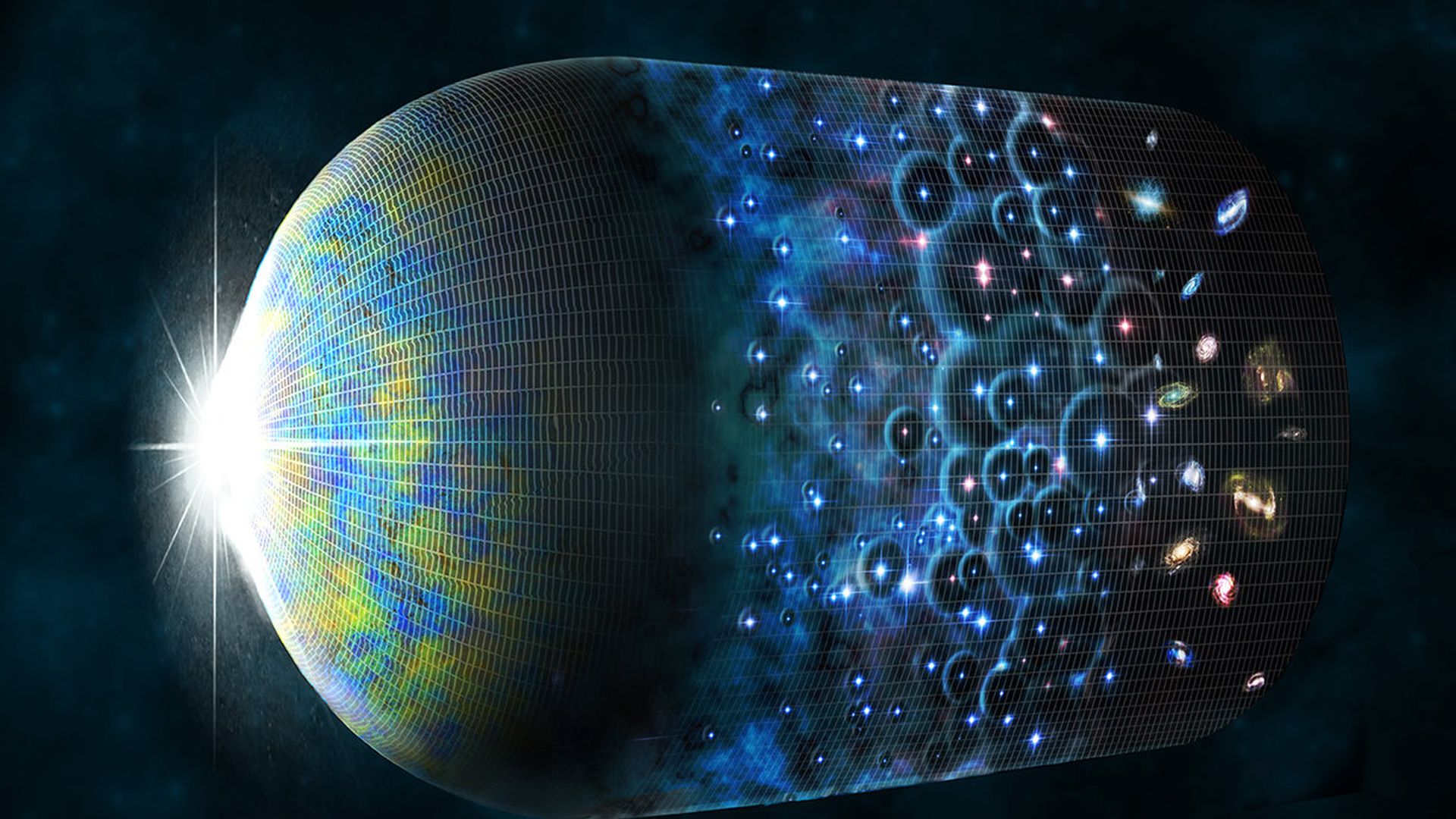
The Universe has an incredible history, and has undergone a tremendous number of changes over time: entirely, as far as we understand it, due to processes that arise solely as a consequence of the laws that govern the workings of nature. If we begin at the start of the hot Big Bang, we find that the Universe wasn’t at all like what we recognize today, but rather was:
- extremely hot and energetic, where the typical particle possessed approximately an octillion (1027) times as much energy as a typical room-temperature particle,
- symmetric between matter and antimatter, where for every quantum of matter that existed, an equal quantum of antimatter also existed,
- incredibly dense, where the average density was somewhere around ~1080 times (or more) denser than the Universe we inhabit today,
- very very uniform, unlike today, where even the greatest densest regions were only ~0.01% denser than average, and where the lowest density regions were only ~0.01% less dense than average,
- and also was very rapidly expanding, where the average distance between particles was increasing rapidly, leading also to a swift loss of energy-per-particle and a rapid cooling on a cosmic scale.
Even though the Universe began almost perfectly uniform, and was filled with all the known quanta (particles, antiparticles, as well as the bosons that are neither matter nor antimatter) in roughly equal amounts, there are two key factors that cannot be ignored when it comes to the Universe’s evolution. One, there are forces (and interactions) that take place between these quanta, and two, these conditions represent an out-of-equilibrium state. As the Universe expands and cools, many of its initial properties begin to rapidly change.
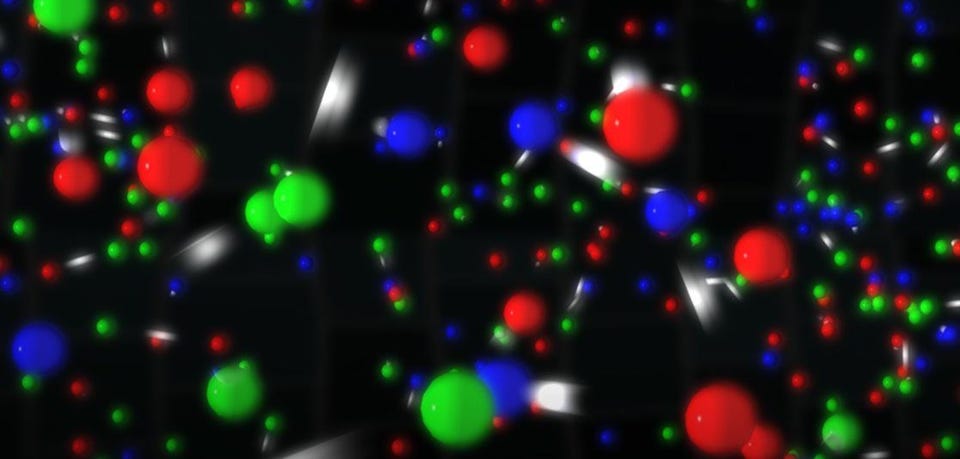
The first change isn’t “clumping” as you might expect from the law of gravity, but rather an evolution in what types of species of particle (or antiparticle) survive. Inherent to every particle (or antiparticle) that exists is a certain amount of energy that can never be extricated from that quantum itself: rest mass energy, or the energy from Einstein’s equation E = mc². As the Universe expands and cools, it cools through a phase known as the electroweak transition, and this triggers an event known as symmetry breaking. The Higgs field transitions from a symmetric to an asymmetric state, the weak nuclear force separates from the electromagnetic force, and the particles of the Standard Model acquire a rest mass. (Also, either during this transition or before it, a slight excess of one extra matter particle for every ~1.6 billion antimatter particles is produced.)
This causes the most unstable particles — the ones with the greatest rest masses and the shortest mean lifetimes — as well as their antiparticle counterparts to decay away. Top quarks (and antiquarks) go away first, followed by W-and-Z bosons and Higgs bosons. Then tau leptons (and antileptons) cease to exist, and then another transition occurs: the QCD phase transition, or the Universe transitioning from a quark-gluon plasma to one that possesses bound states of quarks and/or antiquarks:
- baryons (combinations of three quarks),
- antibaryons (combinations of three antiquarks),
- and mesons (quark-antiquark pairs).
This “zoo” of particles only exists for a brief while, and as the Universe expands and cools, the heaviest of these combinations decays away the fastest.
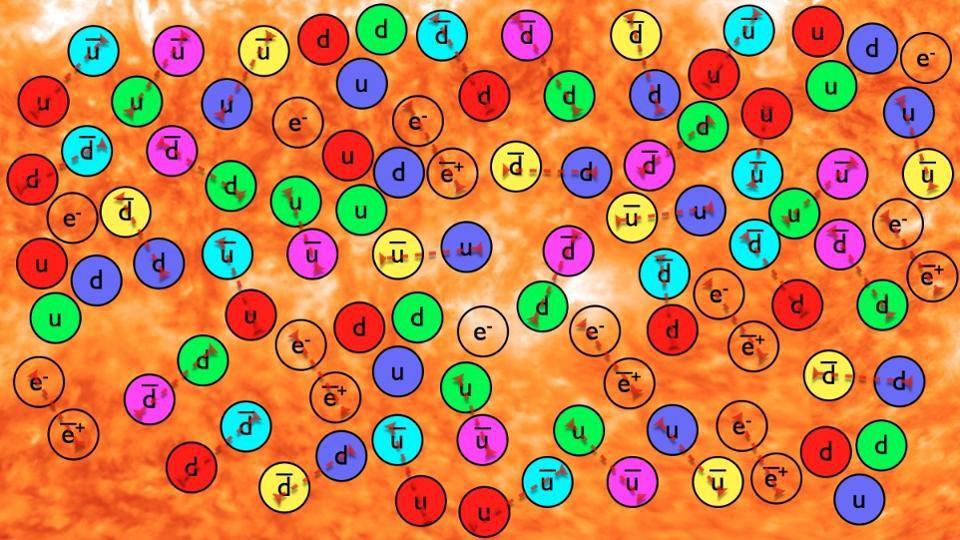
In a certain sense, you could argue that the Universe “selects against” certain species of particles: both fundamental particles (quarks and leptons, as well as bosons) as well as composite ones (baryons, antibaryons, and mesons), as only a few member species will ultimately survive. Composite particles that contain bottom, charm, and/or strange quarks (or antiquarks) decay away, leaving only those particles that contain up-and-down quarks alone: protons, neutrons, antiprotons, and antineutrons, as well as pions. Once the Universe cools sufficiently so that it becomes impossible to produce proton-antiproton (or neutron-antineutron) pairs via collisions with enough energy to form them (again, via E = mc²), then protons and antiprotons and neutrons and antineutrons annihilate away, leaving only that small excess of protons and neutrons.
At around the same time, the last of the pions (the longest-lived meson species) decay into muons and antimuons, and then the muons and antimuons annihilate and/or decay away, leaving electron-positron pairs as the last remaining reserve of large amounts of antimatter behind. Electrons and positrons, as well as neutrinos and antineutrinos, play a role in favoring the conversion of neutrons into protons versus the conversion of protons into neutrons. Then:
- when the Universe is about one second old, the weak interactions freeze out, and proton-neutron interconversion ceases,
- when the Universe is about three seconds old, electrons and positrons annihilate away, leaving only a tiny excess of electrons (which balances the number of protons, maintaining the electric neutrality of the Universe),
- and finally, when the Universe is a few minutes old, nuclear fusion reactions can stably proceed, producing the light atomic nuclei.
In only ~20 minutes, we went from an almost-perfectly uniform bath of particles and antiparticles to a sea of much-lower-energy photons and neutrinos (and antineutrinos) speckled with atomic nuclei and enough electrons to keep the Universe electrically neutral.
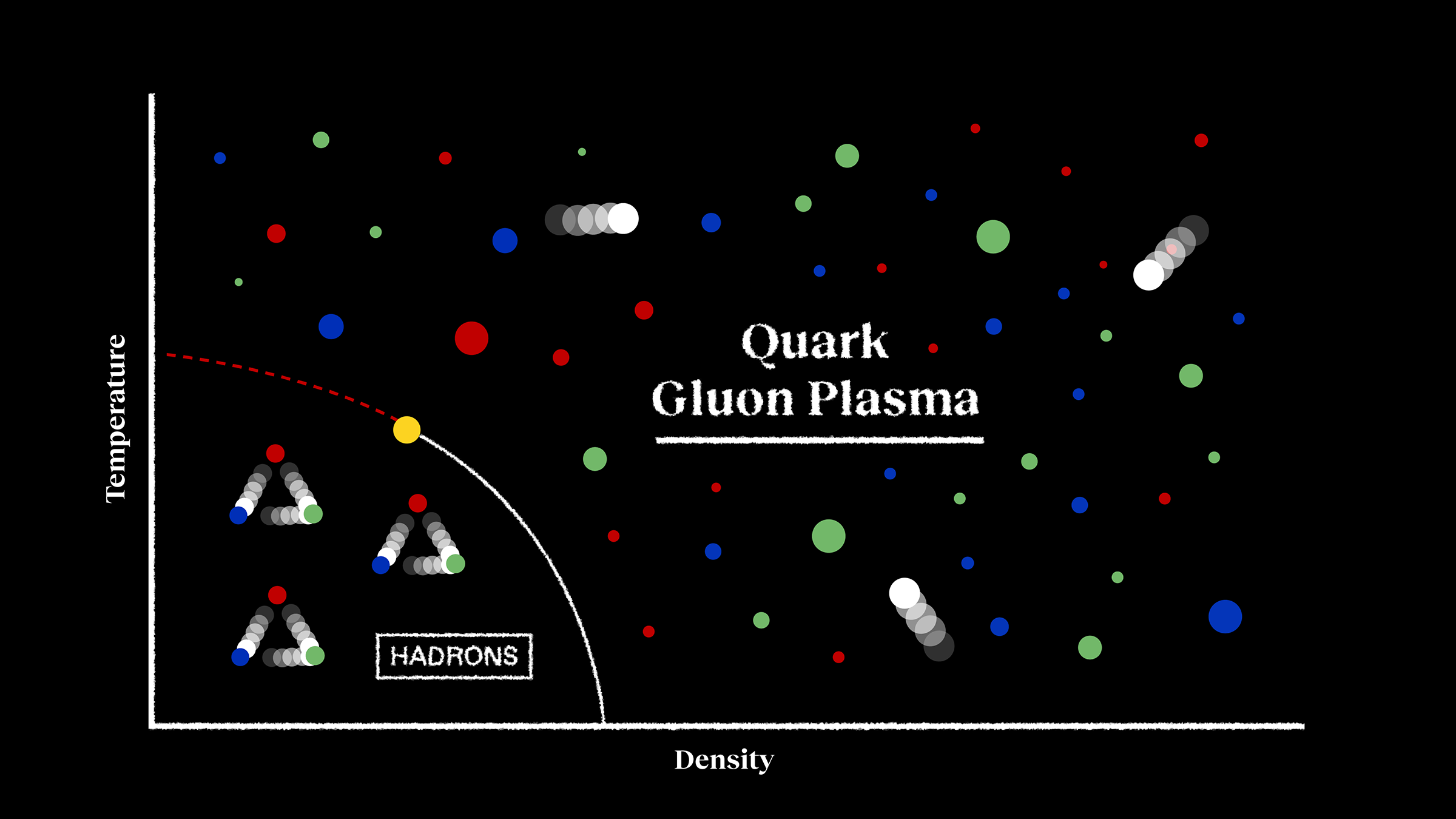
Now, let’s take a look at how these processes occurred, and what the physical reason was behind this sort of cosmic evolution. Although I myself am often guilty of saying that “physics doesn’t answer questions of why something occurs,” it can explain, for lack of a better word, why the Universe became filled with protons, nuclei containing both protons-and-neutrons, electrons, and only a low-energy bath of photons and neutrinos (and antineutrinos) after beginning with a tremendously diverse array of particles and antiparticles. The key concept is stability, and a tendency for things to transition toward the most stable, lowest-energy states.
Consider the following question: why, for example, is the proton stable? Why doesn’t the proton decay into anything else?
The answer is twofold. First, because the proton is the lightest, lowest-mass baryon in all of existence. Made up of two up quarks (the lightest quark species) and one down quark (the second-lightest quark species), the proton has a rest mass of 1.673 × 10-27 kg (or 938.272 MeV/c²), which means it would require additional amounts of energy to transform it into any other type of baryon. The neutron, the next-lightest baryon, has a rest mass of 1.675 × 10-27 kg (or 939.565 MeV/c²), and a free neutron will decay into a proton, an electron, and an electron antineutrino with a mean lifetime of around 15 minutes. Once there is no longer a sufficient amount of energy to create these heavier baryons, spontaneously, only the lightest, most stable ones will survive.
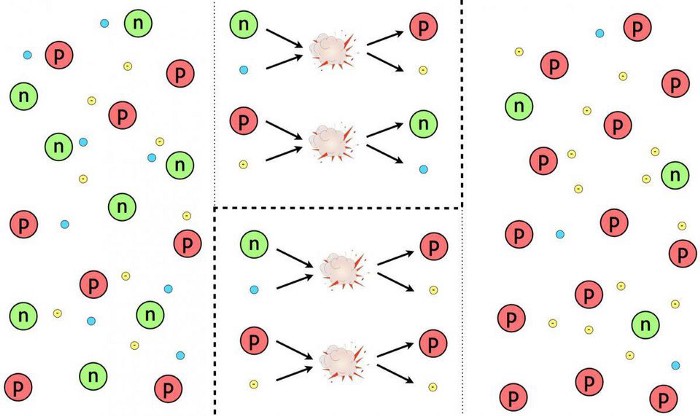
There are also conservation laws and symmetries at play in our Universe: fundamental ones as well as approximate ones that hold under all conventional, experimental circumstances ever probed by humanity. Baryon number, or the total number of baryons minus the total number of antibaryons, is one such conserved quantity, and lepton number, or the total number of leptons minus the total number of antileptons, is another. Although you might imagine that a proton could decay into, say, a pion plus a lepton (a neutral pion plus an electron, or a positively charged pion plus a neutrino, for example), those reactions would violate both the conservation of baryon number and also the conservation of lepton number.
That’s it. We only need to consider the dual facts that:
- the Universe selects for more energetically favorable configurations, and selects against more energy-costly configurations,
- and that there are conserved quantities and symmetries that must be obeyed and cannot be broken, at least conventionally,
to explain how the overwhelming majority of cosmic evolution occurs, particularly during the early stages of our Universe. Protons and neutrons are the most stable baryons, so they’re the only ones that survive the hot, dense, early stage. Electrons are the most stable charged leptons, so they’re the only ones that survive. Protons and electrons don’t decay into lighter particles, because there are conserved quantities (baryon number, lepton number, electric charge, etc.) that cannot be violated. And some neutrons persist because the bound states of neutrons-and-protons, together, are more stable than isolated protons on their own.
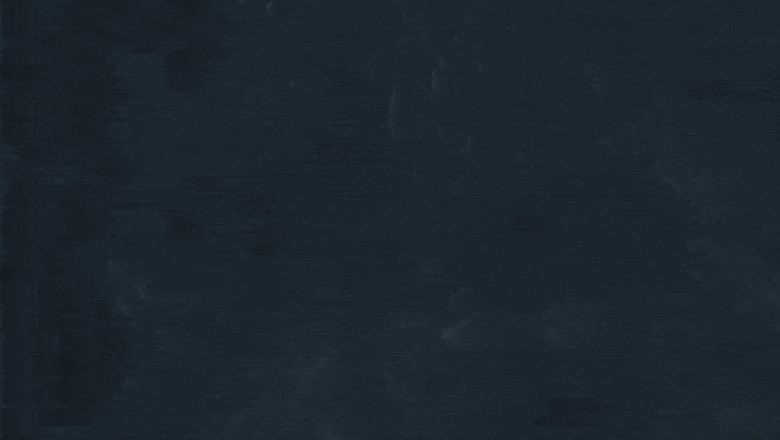
Similarly, as the Universe continues to expand and cool, there are more changes that occur. When the photon energy drops to sufficiently low values, protons (and other atomic nuclei) can combine with electrons to form neutral atoms: a more stable, lower-energy state than simply an ionized plasma of free protons (and atomic nuclei) along with free electrons. Gravitational collapse begins to occur, leading to the formation of cosmic structure, because a gravitationally collapsed collection of masses is more energetically stable than a uniform collection of masses. In other words, things evolve toward an equilibrium state, physically, in nature, and equilibrium is characterized by whatever state has the lowest amount of free energy.
However, it’s often the case that what applies globally can be violated locally.
When masses gravitationally collapse, they lead to high densities at the centers, or cores, of these large collections of mass. Because the matter in our Universe is made primarily out of atoms — or the ingredients of atoms, like protons, neutrons, and electrons — we can’t simply treat our Universe as only being subject to the law of gravity. There are other laws and other forces as well: there are electromagnetic interactions, as the electron and the proton have electric charges, and there are nuclear interactions, as protons and/or neutrons can either fuse together to make heavier elements, or heavy nuclei can be split apart through either fission reactions or the process of spallation. When enough mass gathers in one region of space, remarkable structures spontaneously arise.
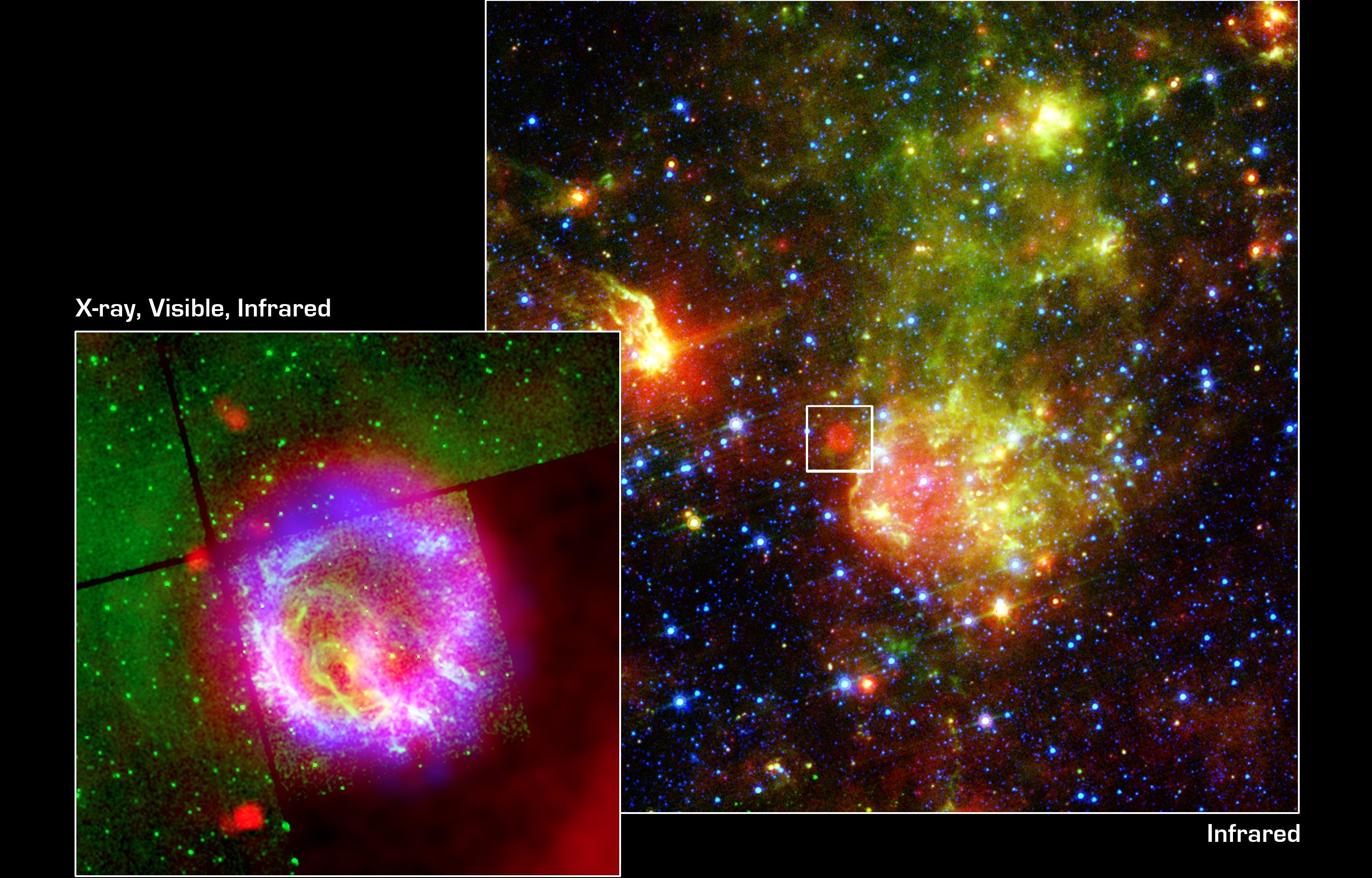
Most prominently, of course, is the entity of a star: a region of space that has accumulated somewhere around ~1056 baryons (protons and/or neutrons) or more in one single location. Bring this amount of mass together, and the central, core temperature will crest above a critical threshold of around ~4 million K: the key temperature at which nuclear fusion between protons begins to spontaneously occur. As the Universe ages and approaches equilibrium on a global scale, the gravitational collapse of matter can trigger the onset of nuclear reactions. Although we talk about the initiation of this process as “star birth” or the ongoing nature of this process as “stars living their lives,” there’s nothing biological about it at all.
What’s happening is that these stars, although they are in fact producing energy through the process of nuclear fusion, are transitioning to a lower-energy state. As light atomic nuclei fuse into heavier atomic nuclei, mass (from the protons and neutrons themselves) is being converted into radiation energy, again via Einstein’s most famous equation, E = mc². The more that fusion occurs, the more the core of these stars becomes filled with more stable, heavier atomic nuclei: nuclei from which less energy, overall, can be extracted. As stars burn through the fuel in their core, they eventually reach the end of the line. Depending on their masses, they will die in either supernova explosions, leaving behind a neutron star or white dwarf, or will die by blowing off their outer layers in a planetary nebula, while their cores contract down to a white dwarf. (In the far future, the lowest mass stars will die by becoming white dwarfs without emitting a planetary nebula, but the Universe is not yet old enough for that to have occurred even once.)
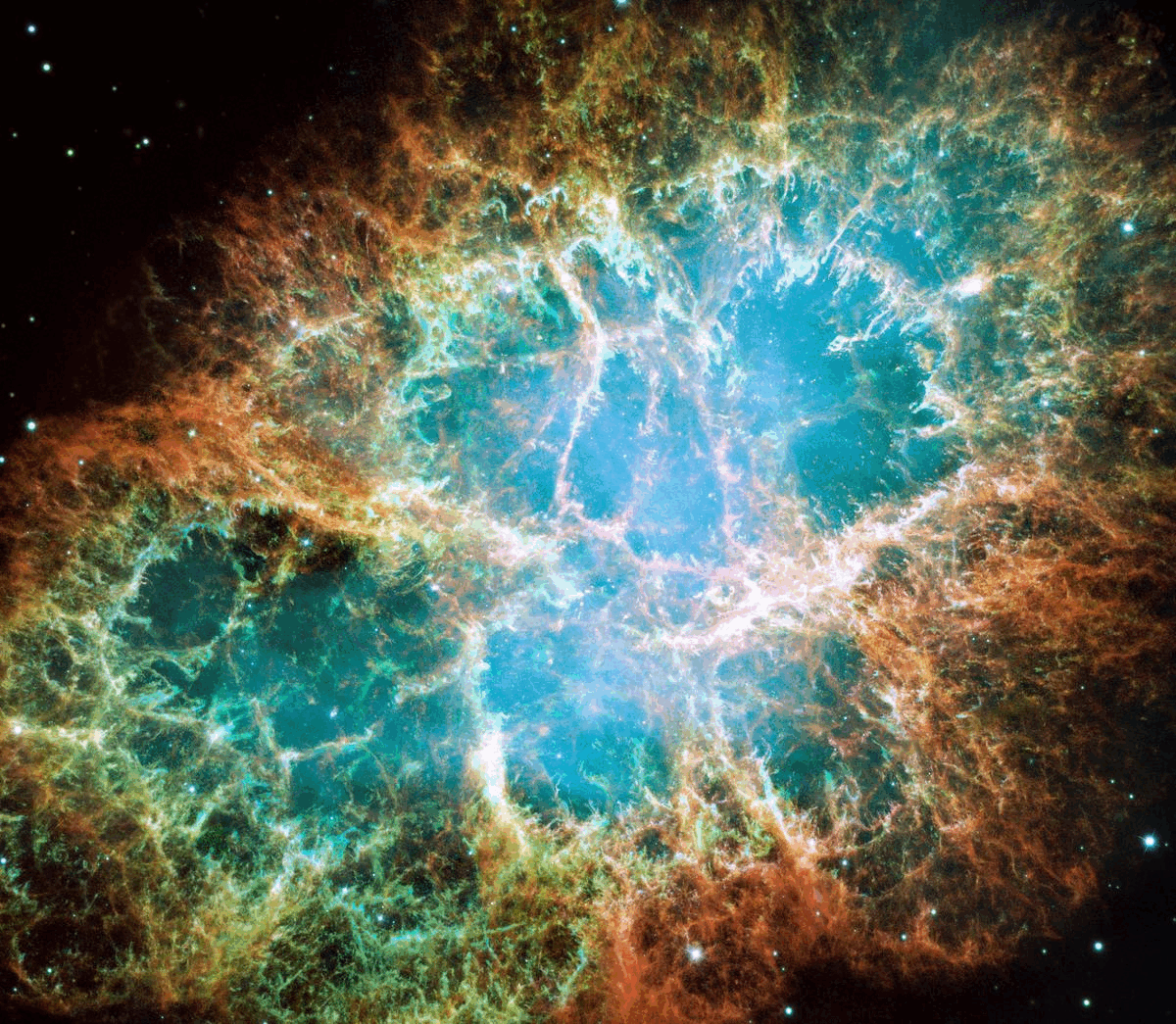
It might not seem obvious, but black holes, neutron stars, and white dwarfs all have less energy, and are more stable, than full-fledged, “living” stars are. They are a more energetically stable end-state, and are known collectively as stellar remnants. However, the processes that lead to them — the ones involving supernovae or the production of a planetary nebula — all return not just hydrogen and helium to the Universe, but large amounts of those heavy elements that were produced inside the stars, both during the “living” phase of the star and also during the end-stages of stellar death. These heavy elements then mix with the atoms in the interstellar medium, giving rise to the opportunity to form next-generation stars with heavier elements, and, as a result, the potential for rocky, solid planets around them.
Even as the Universe tends toward a progressively lower-energy state, and to a state of ever-increasing entropy, overall, there are local places that can receive energy and where physical processes can leverage that inputted energy to do work, and work against the overall increase of entropy. Planet Earth has been one such place where that has occurred: driven by energy sources, such as hydrothermal vents in the ocean or where direct sunlight strikes Earth’s surface, atoms and molecules can be excited to higher-energy states, and that energy can be used to initiate, sustain, and continuously power the activity we know as life processes. “Feeding off” of the energy outputted by large, massive objects that are tending toward equilibrium, biological processes can, on a very small scale compared to the cosmos, create extremely ordered entities, including ourselves.
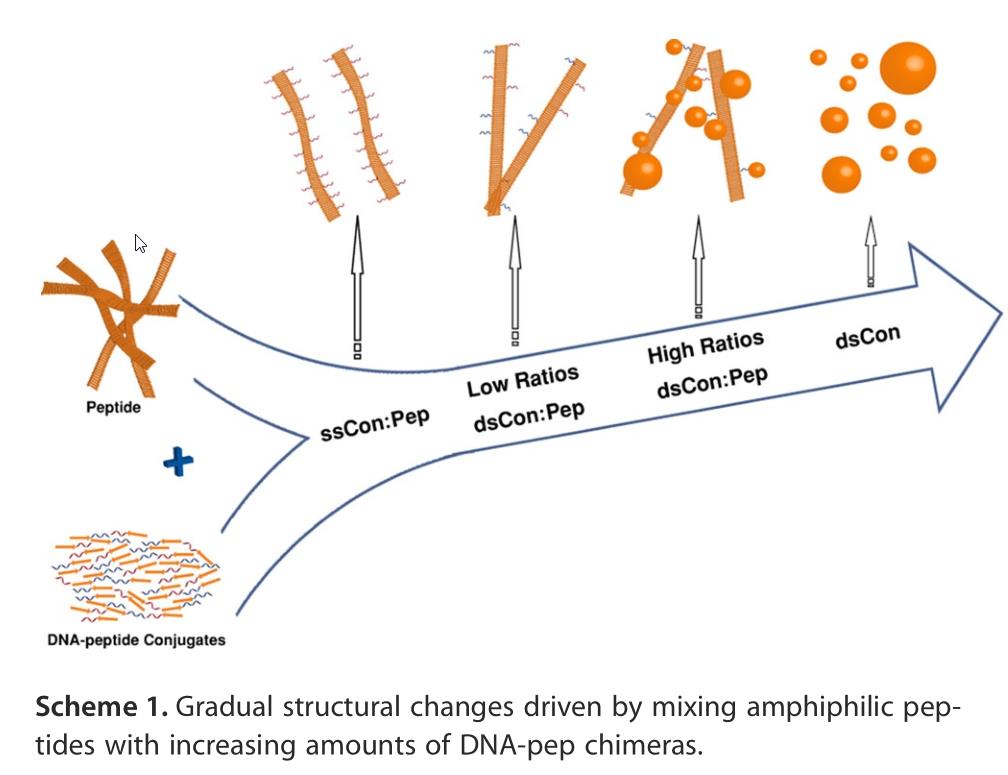
Eventually, all of this will come to an end. Even though our Universe will continue to have new stars for trillions and plausibly even quadrillions of years, eventually, they will all die. Eventually, mutual interactions between stars and stellar remnants within a galaxy will lead to their ejections or to their absorption by black holes. On long enough timescales, even the most massive black holes will decay away, leading to a “heat death” of the Universe, or an equilibrium, lowest-energy state for everything within the observable Universe. Once this state has been achieved, no further energy can be extracted from any known source, and there will be no further meaningful cosmic evolution.
Governed solely by a tendency for nature to take paths toward the lowest-energy, most-equilibrium states, and restricted by the symmetries and conservation laws that govern the Universe, a star-and-galaxy rich cosmos, littered with stellar and planetary systems where biological processes can take place, emerges naturally from a hot, dense, expanding, almost-perfectly-uniform initial state. In this sense, cosmic evolution is inevitable, and higher-energy states are selected against whereas lower-energy states are selected for. This is as far as one can reasonably stretch the analogy, but when it comes to the question of evolution and natural selection, even on solely physical grounds, it’s difficult to fathom how the Universe, while still obeying the laws of nature that we’re familiar with, could ever have done anything else.
Send in your Ask Ethan questions to startswithabang at gmail dot com!