Why a monkey can lose its visual cortex without going blind
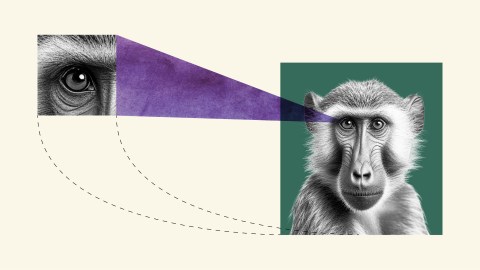
- In Sentience: The Invention of Consciousness, theoretical psychologist Nicholas Humphrey explores the evolutionary history of consciousness.
- This excerpt of the book includes Humphrey’s firsthand account of animal experiments he conducted to study how mammalian brains process visual information.
- The visual cortex isn’t the only system that can make sense of complex visual information.
I began research for a PhD in psychology in 1964. My supervisor now was Larry Weiskrantz, a man in marked contrast to Brindley, not so fiercely clever, but much kinder to himself and others.
Larry was born in New York to immigrants from Germany. When he was aged six, his father suddenly died, and his family lost their sole source of income. His mother had no choice but to send Larry to a free boarding school for ‘poor male white orphans’. Within this school and, as Larry would say, ‘only in America’, he flourished, progressing by scholarships to Harvard, Oxford, and Cambridge.
In Cambridge he began a program of research into the brain mechanisms underlying vision in monkeys. A big question was the role of the cerebral cortex in vision. In all mammals, there are two brain pathways for processing information from the eyes, an evolutionarily ancient one and a more modern one. The ancient pathway, which is also present in vertebrates such as fish and frogs whose brains do not have a cortex, runs from the eyes to the optic tectum in the midbrain. The other pathway, that evolved in the mammalian line, runs to the primary visual cortex.
Larry had been studying the effects of surgically removing the visual cortex from a monkey’s brain. His research at this point had largely confirmed the conventional wisdom: that the operation left the monkey for all practical purposes completely blind. True, the monkey could still learn to choose between cards of different brightness and between a uniform grey card and a checkerboard pattern, but it was quite unable to discriminate the position or shape of objects. ‘The simplest hypothesis concerning the capacity of this monkey is that it responded only to the integral of all retinal ganglionic activity. There is no suggestion it could respond to the distribution of variation.’ In other words, it seemed that monkeys’ eyes were simply serving as light buckets without providing any information about the spatial pattern on the retina.
This was in line with earlier findings. Yet, a question hung over it. The monkey’s midbrain visual system was still intact. Fish and frogs can see just fine using the optic tectum. Why should the monkey become so incapacitated visually after the operation?
It was agreed that I should embark on a study of single nerve cells in the monkey’s optic tectum (also known as the superior colliculus) to see just what kinds of visual information this secondary system might be capable of processing. Since no one else in our lab knew the techniques of recording from single cells, Larry sent me to Edinburgh for a couple of months to be shown the ropes by the renowned neuroscientist David Whitteridge.
Whitteridge took me under his wing. He taught me how to make fine-tipped needle electrodes for recording the electrical activity of cells in the brain. Then, he demonstrated how to surgically open up a hole in the skull of an anesthetized cat and insert the needle through the brain to just the right position in the visual system close to a responsive nerve cell. When the cell fired, the discharge was picked up by the electrode and amplified so that we could hear it on a loudspeaker.
Back in Cambridge, I adapted these techniques to recording from cells in the superior colliculus in monkeys. The anesthetized monkey was positioned in front of a screen on which I could move small black or luminous targets. I arranged that the position of the target was displayed as a spot on an oscilloscope and linked the brightness of the spot to the cell’s response so that it lit up only when a spike occurred. This meant a picture would emerge on the oscilloscope of the cell’s receptive field—that’s to say the area of space that fell within the cell’s ‘field of view’.
My job was to find what kind of visual stimulus would excite the cell I was recording from. It turned out that cells responded best to moving targets, crossing the screen in any direction, at about 10 degrees a second. Cells near the surface of the colliculus had very small receptive fields, meaning they could potentially pinpoint exactly where the target was situated; but as the electrode went deeper the fields became much larger, meaning they were indifferent to the target’s exact location. Figure 5.1 shows results for a range of different cells.
These were new and interesting findings. They showed that the surface layers of the colliculus could be relaying information to the rest of the brain about a target’s position and so might in principle be able to support spatial vision—even if this didn’t fit with the behavior of Weiskrantz’s operated monkeys. This would be an important lead to follow up. But while I was doing the experiments, I confess the nice ‘results’ I was getting were not always what most concerned me. I look back now and my heart misses a beat.
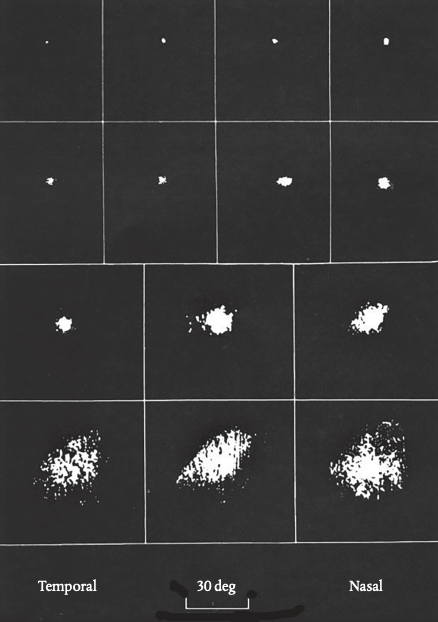
I was a twenty-three-year-old student working alone, often deep into the night, in a blacked-out room in a deserted building. There was an anesthetized monkey tied to a chair. The only light came from the targets moving across the screen and the winking oscilloscope; the only sound was the sporadic crackle of spikes from the loudspeaker. The animal would be put to sleep permanently when I was done with it. The brain cells I was listening to were ‘seeing’ for the last time. In this borderline situation, strange thoughts swam through my mind.
If the animal were awake, it would be having a visual sensation as the stimulus moved across its retina. But because sensations are private, no one would be able to tell this from the outside. Now, however, some part at least of this private experience had become externalized: the animal’s response to light was showing up on the oscilloscope and activating the loudspeaker. It was as if the animal was expressing how it felt about the stimulus out loud—growling, say, or purring as the light stroked its retina. And here was I, listening in. But if I could listen to how the monkey felt about the visual stimulus, perhaps the monkey could be listening too?
Next, poetry took over. I had already come across certain cells that responded to a moving target with a series of bursts of spikes rather than a regular string: whoosh! a pause, then whoosh! (See Figure 5.2.) What was this about? On a hunch, the next time I found a cell responding in this odd way, I covered the monkey’s ears with a bandage. The whooshing stopped, and the cell responded to the target with a steady discharge. Then I uncovered the ears and turned down the volume of the speaker so that I could only just hear it. Again, no whooshing.
This must have been what’s called a ‘multi-modal’ cell—a cell that had input from the ears as well as the eyes. I had found other examples, although they were relatively rare. Could it be that, when the volume of the speaker was turned up, the cell was first responding to the visual target with a burst of spikes, then responding to the sound these gave rise to with more spikes, and then to the sound these gave rise to? The result would be positive feedback, creating a brief surge in activity that would exhaust itself. Whoo. . .sh! To test this, without a visual target, I clapped my hands. Sure enough, the cell responded with a whoosh to the sound of the clap. The effect of the auditory feedback was that the cell’s response to any stimulus was getting drawn out in time, producing a kind of afterglow.
The set-up in the lab was, of course, completely artificial. But this unexpected phenomenon gave me the seed of an idea. We tend to think of sensations as experiences that are impressed on us from the outside. But suppose our sensations actually originate as an active bodily response to the stimulus, like the signals sent to the speaker, and that we only become aware of this when we monitor our own response by a kind of listening in? Could this—and the feedback loops that would easily follow—be what gives sensations the thick, expressive quality that we find so wonderful? This idea would later take root in a theory of qualia. But that was still to come.
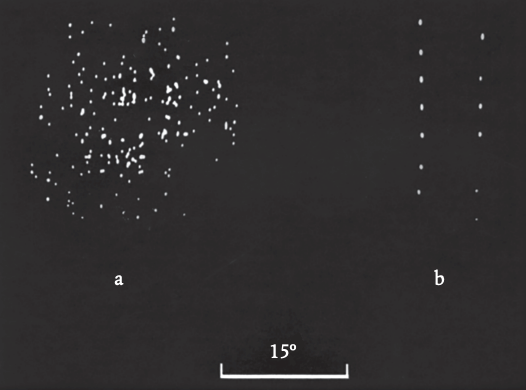
I may say, I didn’t much like doing these experiments. It’s not that I doubted their scientific value. These were the first recordings ever made from the superior colliculus of monkeys (and the 1968 paper where I described them has been cited several hundred times). Nor was it that I thought experiments on living animals were wrong in principle. The monkeys were anesthetized throughout and didn’t suffer. Nonetheless, there was no denying that what I was doing had a worrying power dimension. It could have been said (no one did, but I thought it) that—to put it bluntly—I was valuing my curiosity about how the monkey’s brain works over the monkey’s interest in enjoying the use of its brain. Of course, I hoped my findings would contribute to the larger project of understanding the neuropsychology of vision in monkeys and in humans, so at least it wouldn’t be idle curiosity. But would they contribute?
The thing that certainly looked promising was the evidence that the cells I was studying were quite capable of relaying information about the location of objects in space. In this respect, the monkey’s superior colliculus clearly did indeed resemble the frog’s optic tectum. In fact, the kinds of stimuli the cells responded to were markedly similar to those described in a famous paper by Jerry Lettvin and colleagues in 1959, entitled ‘What the Frog’s Eye Tells the Frog’s Brain’.
After removal of the visual cortex in a monkey, this relatively primitive visual pathway could still be operational. In which case, the monkey might retain at least a frog-like capacity for spatial vision. Yet, Weiskrantz’s research seemed to prove not. I began to wonder. Could Weiskrantz have been missing something? Maybe something that was hiding, not in plain sight, but in some other kind of sight entirely