Ask Ethan: If Matter Is Made Of Point Particles, Why Does Everything Have A Size?
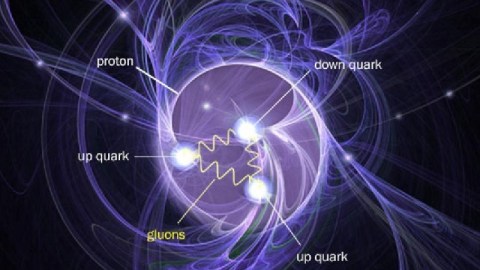
Everything is made of quarks, leptons, photons, and gluons, yet everything comes with a finite, non-zero size.
“There’s something about sitting alone in the dark that reminds you how big the world really is, and how far apart we all are. The stars look like they’re so close, you could reach out and touch them. But you can’t. Sometimes things look a lot closer than they are.” –Kami Garcia
The big idea of atomic theory is that, at some smallest, fundamental level, the matter that makes up everything can be divided no further. Those ultimate building blocks would be literally ἄ-τομος, or un-cuttable. As we’ve gone down to progressively smaller scales, we’ve found that molecules are made of atoms, which are made of protons, neutrons, and electrons, and that protons and neutrons can be further split into quark and gluons. Yet even though quarks, gluons, electrons, and more appear to be truly point-like, all the matter made out of them has a real, finite size. Why is that? That’s what Brian Cobb wants to know:
Many sources state that quarks are point particles… so one would think that objects composed of them — in this instance, neutrons — would also be points. Is my logic flawed? Or would they be bound to each other in such a way that they would cause the resulting neutron to have angular size?
Let’s take a journey down to the smallest scales, and find out what’s truly going on.
If we take a look at matter, things behave similar to how we expect they should, in the macroscopic world, down to about the size of molecules: nanometer (10–9 meter) scales. On smaller scales than that, the quantum rules that govern individual particles start to become important. Single atoms, with electrons orbiting a nucleus, come in at about the size of an Angstrom: 10–10 meters. The atomic nucleus itself, made up of protons and neutrons, is 100,000 times smaller than the atoms in which they are found: a scale of 10–15 meters. Within each individual proton or neutron, quarks and gluons reside. While molecules, atoms, and nuclei all have sizes associated with them, the fundamental particles they’re made out of — quarks, gluons, and electrons — are truly point-like.
The way we determine whether something is point-like or not is simply to collide whatever we can with it at the highest possible energies, and to look for evidence that there’s a composite structure inside. In the quantum world, particles don’t just have a physical size, they also have a wavelength associated with them, determined by their energy. Higher energy means smaller wavelength, which means we can probe smaller and more intricate structures. X-rays are high-enough in energy to probe the structure of atoms, with images from X-ray diffraction and crystallography shedding light on what molecules look like and how individual bonds look.
At even higher energies, we can get even better resolution. Particle accelerators could not only blast atomic nuclei apart, but deep inelastic scattering revealed the internal structure of the proton and neutron: the quarks and gluons lying within. It’s possible that, at some point down the road, we’ll find that some of the particles we presently think are fundamental are actually made of smaller entities themselves. At the present point, however, thanks to the energies reached by the LHC, we know that if quarks, gluons, or electrons aren’t fundamental, their structures must be smaller than 10–18to 10–19 meters. To the best of our knowledge, they’re truly points.
So how, then, are the things made out of them largerthan points? It’s the interplay of (up to) three things:
- Forces,
- Particle properties,
- and Energy.
The quarks that we know don’t just have an electric charge, but also (like the gluons) have a color charge. While the electric charge can be positive or negative, and while like charges repel while opposites attract, the force arising from the color charges — the strong nuclear force — is always attractive. And it works, believe it or not, much like a spring does.
When two color-charged objects are close together, the force between them drops away to zero, like a coiled spring that isn’t stretched at all. When quarks are close together, the electrical force takes over, which often leads to a mutual repulsion. But when the color-charged objects are far apart, the strong force gets stronger. Like a stretched spring, it works to pull the quarks back together. Based on the magnitude of the color charges and the strength of the strong force, along with the electric charges of each of the quarks, that’s how we arrive at the size of the proton and the neutron: where the strong and electromagnetic forces roughly balance.
On slightly larger scales, the strong force holds protons and neutrons together in an atomic nucleus, overcoming the electrostatic repulsion between the individual protons. This nuclear force is a residual effect of the strong nuclear force, which only works over very short distances. Because individual protons and neutrons themselves are color-neutral, the exchange is mediated by virtual, unstable particles known as pions, which explains why nuclei beyond a certain size become unstable; it’s too difficult for pions to be exchanged across larger distances. Only in the case of neutron stars does the addition of gravitational binding energy suppress the nucleus’ tendency to rearrange itself into a more stable configuration.
And on the scale of the atom itself, the key is that the lowest-energy configuration of any electron bound to a nucleus isn’t a zero-energy state, but is actually a relatively high-energy one compared to the electron’s rest mass. This quantum configuration means that the electron itself needs to zip around at very high speeds inside the atom; even though the nucleus and the electron are oppositely charged, the electron won’t simply hit the nucleus and remain at the center. Instead, the electron exists in a cloud-like configuration, zipping and swirling around the nucleus (and passing through it) at a distance that’s almost a million times as great as the size of the nucleus itself.
There are some fun caveats that allow us to explore how these sizes change in extreme conditions. In extremely massive planets, the atoms themselves begin to get compressed due to large gravitational forces, meaning you can pack more of them into a small space. Jupiter, for example, has three times the mass of Saturn, but is only about 20% larger in size. If you replace an electron in a hydrogen atom with a muon, an unstable electron-like particle that has the same charge but 206 times the mass, the muonic hydrogen atom will be only 1/206th the size of normal hydrogen. And a Uranium atom is actually larger in size than the individual protons-and-neutrons would be if you packed them together, due to the long-range nature of the electrostatic repulsion of the protons, compared to the short-range nature of the strong force.
By having different forces at play of different strengths, you can build a proton, neutron, or other hadron of finite size out of point-like quarks. By combining protons and neutrons, you can build nuclei of larger sizes than their individual components, bound together, would give you. And by binding electrons to the nucleus, you can build a much larger structure, all owing to the fact that the zero-point energy of an electron bound to an atom is much greater than zero. In order to get a Universe filled with structures that take up a finite amount of space and have a non-zero size, you don’t need anything more than zero-dimensional, point-like building blocks. Forces, energy, and the quantum properties inherent to particles themselves are more than enough to do the job.
Submit your Ask Ethan questions to startswithabang at gmail dot com!
Ethan Siegel is the author of Beyond the Galaxy and Treknology. You can pre-order his third book, currently in development: the Encyclopaedia Cosmologica.