The only way to beat the speed of light
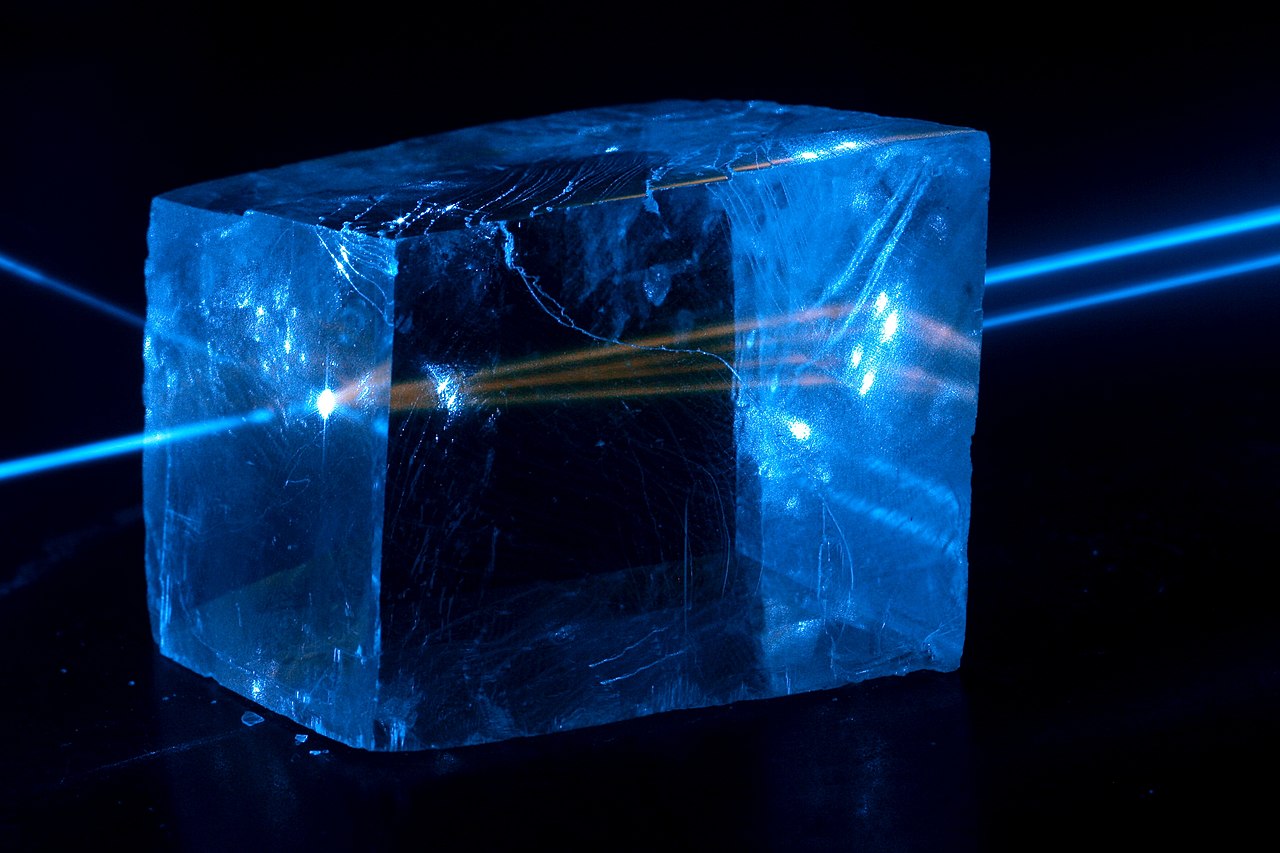
- There’s an ultimate speed limit at which anything can travel through the fabric of space: the speed of light in a vacuum.
- At 299,792,458 m/s, it’s the ultimate cosmic speed limit, and the speed at which all massless particles must travel through empty space.
- But as soon as you’re not in a vacuum any longer, even light itself slows down. This gives rise to the one-and-only way to beat the speed of light: by sending it through a medium.
In our Universe, there are a few rules that everything must obey. Energy, momentum, and angular momentum are always conserved whenever any two quanta interact. The physics of any system of particles moving forward in time is identical to the physics of that same system reflected in a mirror, with particles exchanged for antiparticles, where the direction of time is reversed. And there’s an ultimate cosmic speed limit that applies to every object: nothing can ever exceed the speed of light, and nothing with mass can ever reach that vaunted speed.
Over the years, people have developed very clever schemes to try to circumvent this last limit. Theoretically, they’ve introduced tachyons as hypothetical particles that could exceed the speed of light, but tachyons are required to have imaginary masses, and do not physically exist. Within General Relativity, sufficiently warped space could create alternative, shortened pathways over what light must traverse, but our physical Universe has no known wormholes. And while quantum entanglement can create “spooky” action at a distance, no information is ever transmitted faster than light.
But there is one way to beat the speed of light: enter any medium other than a perfect vacuum. Here’s the physics of how it works.
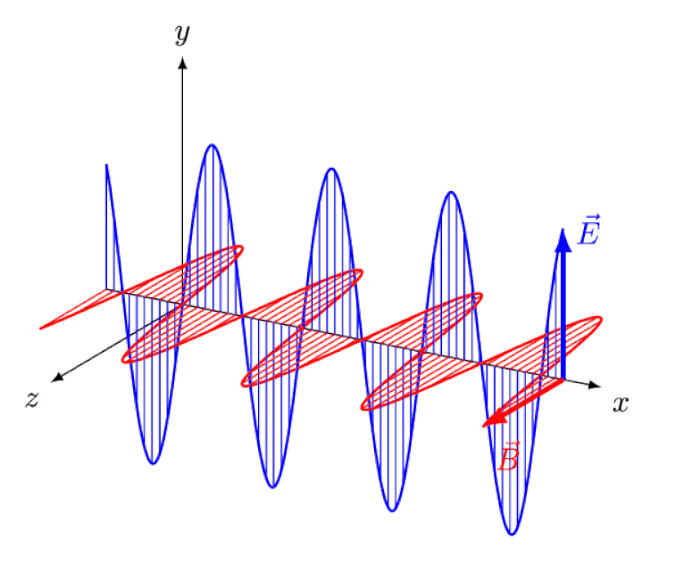
Light, you have to remember, is an electromagnetic wave. Sure, it also behaves as a particle, but when we’re talking about its propagation speed, it’s far more useful to think of it not only as a wave, but as a wave of oscillating, in-phase electric and magnetic fields. When it travels through the vacuum of space, there’s nothing to restrict those fields from traveling with the amplitude they’d naturally choose, defined by the wave’s energy, frequency, and wavelength. (Which are all related.)
But when light travels through a medium — that is, any region where electric charges (and possibly electric currents) are present — those electric and magnetic fields encounter some level of resistance to their free propagation. Of all the things that are free to change or remain the same, the property of light that remains constant is its frequency as it moves from vacuum to medium, from a medium into vacuum, or from one medium to another.
If the frequency stays the same, however, that means the wavelength must change, and since frequency multiplied by wavelength equals speed, that means the speed of light must change as the medium you’re propagating through changes.
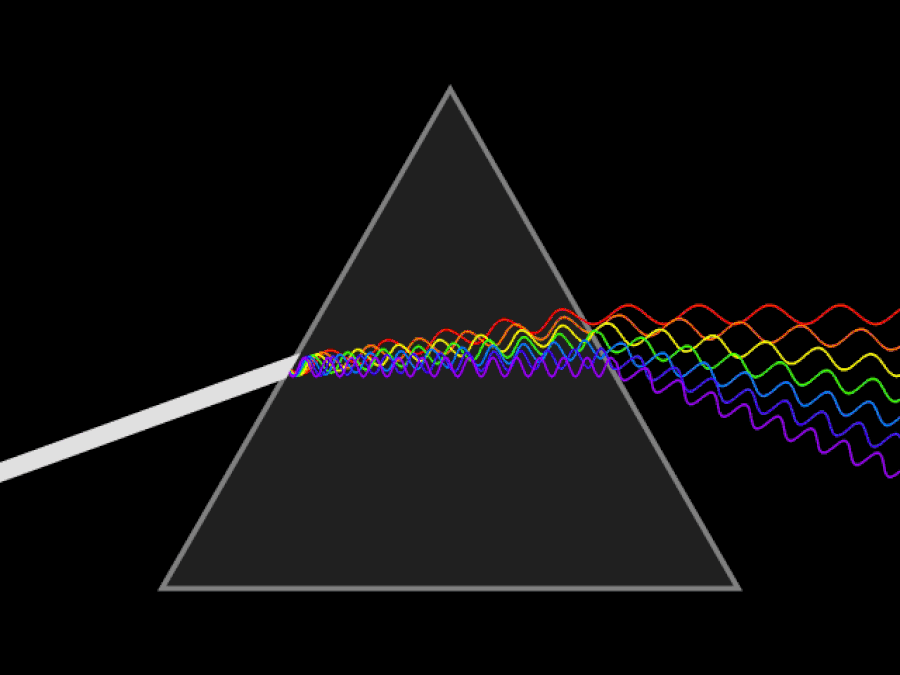
One spectacular demonstration of this is the refraction of light as it passes through a prism. White light — like sunlight — is made up of light of a continuous, wide variety of wavelengths. Longer wavelengths, like red light, possess smaller frequencies, while shorter wavelengths, like blue light, possess larger frequencies. In a vacuum, all wavelengths travel at the same speed: frequency multiplied by wavelength equals the speed of light. The bluer wavelengths have more energy, and so their electric and magnetic fields are stronger than the redder wavelength light.
When you pass this light through a dispersive medium like a prism, all of the different wavelengths respond slightly differently. The more energy you have in your electric and magnetic fields, the greater the effect they experience from passing through a medium. The frequency of all light remains unchanged, but the wavelength of higher-energy light shortens by a greater amount than lower-energy light.
As a result, even though all light travels slower through a medium than vacuum, redder light slows by a slightly smaller amount than blue light, leading to many fascinating optical phenomena, such as the existence of rainbows as sunlight breaks into different wavelengths as it passes through water drops and droplets.
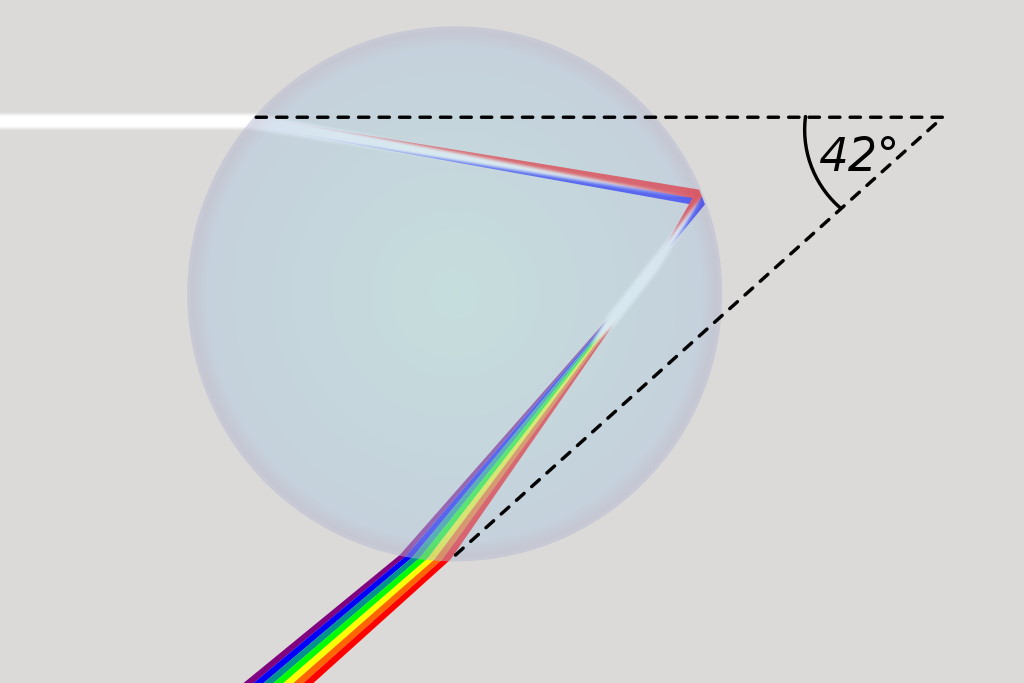
In the vacuum of space, however, light has no choice — irrespective of its wavelength or frequency — but to travel at one speed and one speed only: the speed of light in a vacuum. This is also the speed that any form of pure radiation, such as gravitational radiation, must travel at, and also the speed, under the laws of relativity, that any massless particle must travel at.
But most particles in the Universe have mass, and as a result, they have to follow slightly different rules. If you have mass, the speed of light in a vacuum is still your ultimate speed limit, but rather than being compelled to travel at that speed, it’s instead a limit that you can never attain; you can only approach it.
The more energy you put into your massive particle, the closer it can move to the speed of light, but it must always travel more slowly. The most energetic particles ever made on Earth, which are protons at the Large Hadron Collider, can travel incredibly close to the speed of light in a vacuum: 299,792,455 meters-per-second, or 99.999999% the speed of light.
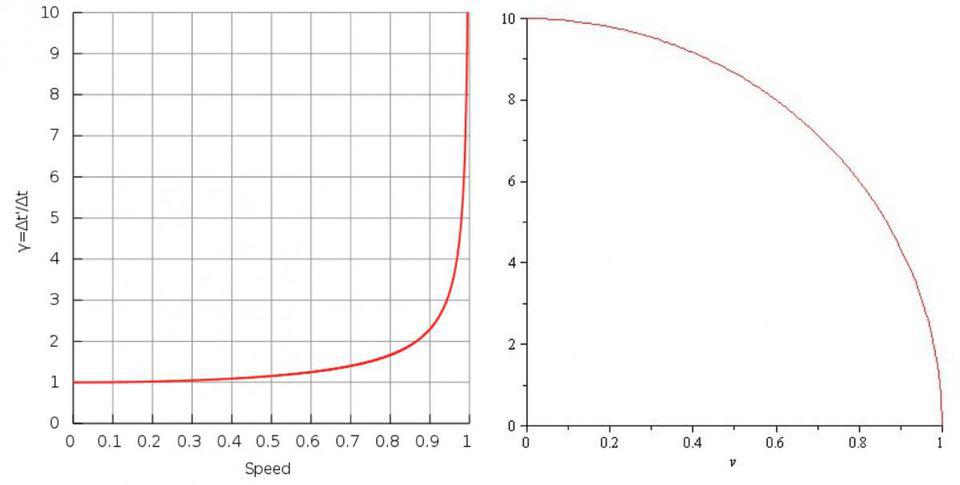
No matter how much energy we pump into those particles, we can only add more “9s” to the right of that decimal place, however. We can never reach the speed of light.
Or, more accurately, we can never reach the speed of light in a vacuum. That is, the ultimate cosmic speed limit, of 299,792,458 m/s is unattainable for massive particles, and simultaneously is the speed that all massless particles must travel at.
But what happens, then, if we travel not through a vacuum, but through a medium instead? As it turns out, when light travels through a medium, its electric and magnetic fields feel the effects of the matter that they pass through. This has the effect, when light enters a medium, of immediately changing the speed at which light travels. This is why, when you watch light enter or leave a medium, or transition from one medium to another, it appears to bend. The light, while free to propagate unrestricted in a vacuum, has its propagation speed and its wavelength depend heavily on the properties of the medium it travels through.

However, particles suffer a different fate. If a high-energy particle that was originally passing through a vacuum suddenly finds itself traveling through a medium, its behavior will be different than that of light.
First off, it won’t experience an immediate change in momentum or energy, as the electric and magnetic forces acting on it — which change its momentum over time — are negligible compared to the amount of momentum it already possesses. Rather than bending instantly, as light appears to, its trajectory changes can only proceed in a gradual fashion. When particles first enter a medium, they continue moving with roughly the same properties, including the same speed, as before they entered.
Second, the big events that can change a particle’s trajectory in a medium are almost all direct interactions: collisions with other particles. These scattering events are tremendously important in particle physics experiments, as the products of these collisions enable us to reconstruct whatever it is that occurred back at the collision point. When a fast-moving particle collides with a set of stationary ones, we call these “fixed target” experiments, and they’re used in everything from creating neutrino beams to giving rise to antimatter particles that are critical for exploring certain properties of nature.

But the most interesting fact is this: particles that move slower than light in a vacuum, but faster than light in the medium that they enter, are actually breaking the speed of light. This is the one and only real, physical way that particles can exceed the speed of light. They can’t ever exceed the speed of light in a vacuum, but can exceed it in a medium. And when they do, something fascinating occurs: a special type of radiation — Cherenkov radiation — gets emitted.
Named for its discoverer, Pavel Cherenkov, it’s one of those physics effects that was first noted experimentally, before it was ever predicted. Cherenkov was studying radioactive samples that had been prepared, and some of them were being stored in water. The radioactive preparations seemed to emit a faint, bluish-hued light, and even though Cherenkov was studying luminescence — where gamma-rays would excite these solutions, which would then emit visible light when they de-excited — he was quickly able to conclude that this light had a preferred direction. It wasn’t a fluorescent phenomenon, but something else entirely.
Today, that same blue glow can be seen in the water tanks surrounding nuclear reactors: Cherenkov radiation.
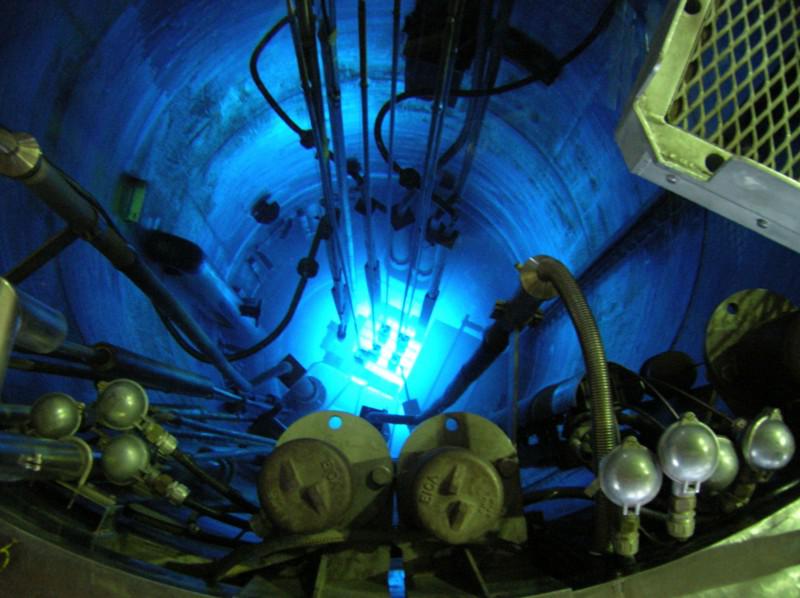
Where does this radiation come from?
When you have a very fast particle traveling through a medium, that particle will generally be charged, and the medium itself is made up of positive (atomic nuclei) and negative (electrons) charges. The charged particle, as it travels through this medium, has a chance of colliding with one of the particles in there, but since atoms are mostly empty space, the odds of a collision are relatively low over short distances.
Instead, the particle has an effect on the medium that it travels through: it causes the particles in the medium to polarize — where like charges repel and opposite charges attract — in response to the charged particle that’s passing through. Once the charged particle is out of the way, however, those electrons return back to their ground state, and those transitions cause the emission of light. Specifically, they cause the emission of blue light in a cone-like shape, where the geometry of the cone depends on the particle’s speed and the speed of light in that particular medium.
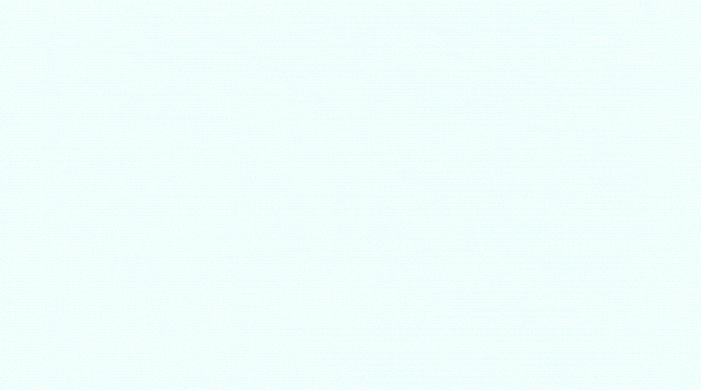
This is an enormously important property in particle physics, as it’s this very process that allows us to detect the elusive neutrino at all. Neutrinos hardly ever interact with matter. However, on the rare occasions that they do, they only impart their energy to one other particle.
What we can do, therefore, is to build an enormous tank of very pure liquid: liquid that doesn’t radioactively decay or emit other high-energy particles. We can shield it very well from cosmic rays, natural radioactivity, and all sorts of other contaminating sources. And then, we can line the outside of this tank with what are known as photomultiplier tubes: tubes that can detect a single photon, triggering a cascade of electronic reactions enabling us to know where, when, and in what direction a photon came from.
With large enough detectors, we can determine many properties about every neutrino that interacts with a particle in these tanks. The Cherenkov radiation that results, produced so long as the particle “kicked” by the neutrino exceeds the speed of light in that liquid, is an incredibly useful tool for measuring the properties of these ghostly cosmic particles.
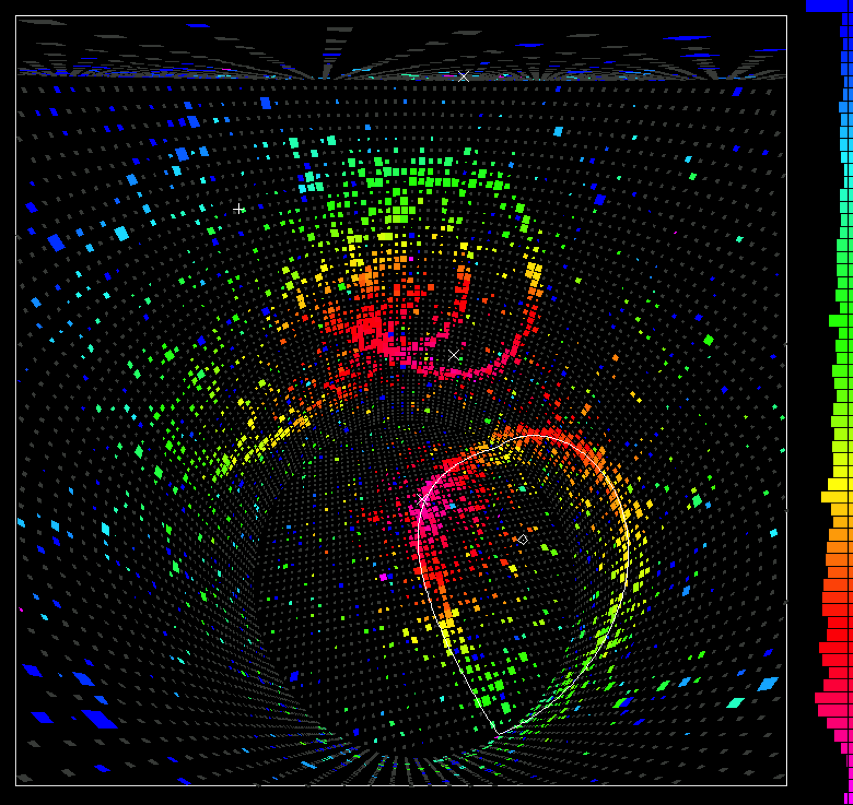
The discovery and understanding of Cherenkov radiation was revolutionary in many ways, but it also led to a frightening application in the early days of laboratory particle physics experiments. A beam of energetic particles leaves no optical signature as it travels through air, but will cause the emission of this blue light if it passes through a medium where it travels faster than light in that medium. Physicists used to close one eye and stick their head in the path of the beam; if the beam was on, they’d see a “flash” of light due to the Cherenkov radiation generated in their eye, confirming that the beam was on. (Needless to say, this process was discontinued with the advent of radiation safety training.)
Still, despite all the advances that have occurred in physics over the intervening generations, the only way we know of to beat the speed of light is to find yourself a medium where you can slow that light down. We can only exceed that speed in a medium, and if we do, this telltale blue glow — which provides a tremendous amount of information about the interaction that gave rise to it — is our data-rich reward. Until warp drive or tachyons become a reality, the Cherenkov glow is the #1 way to go!