The biggest questions about the Universe’s beginning
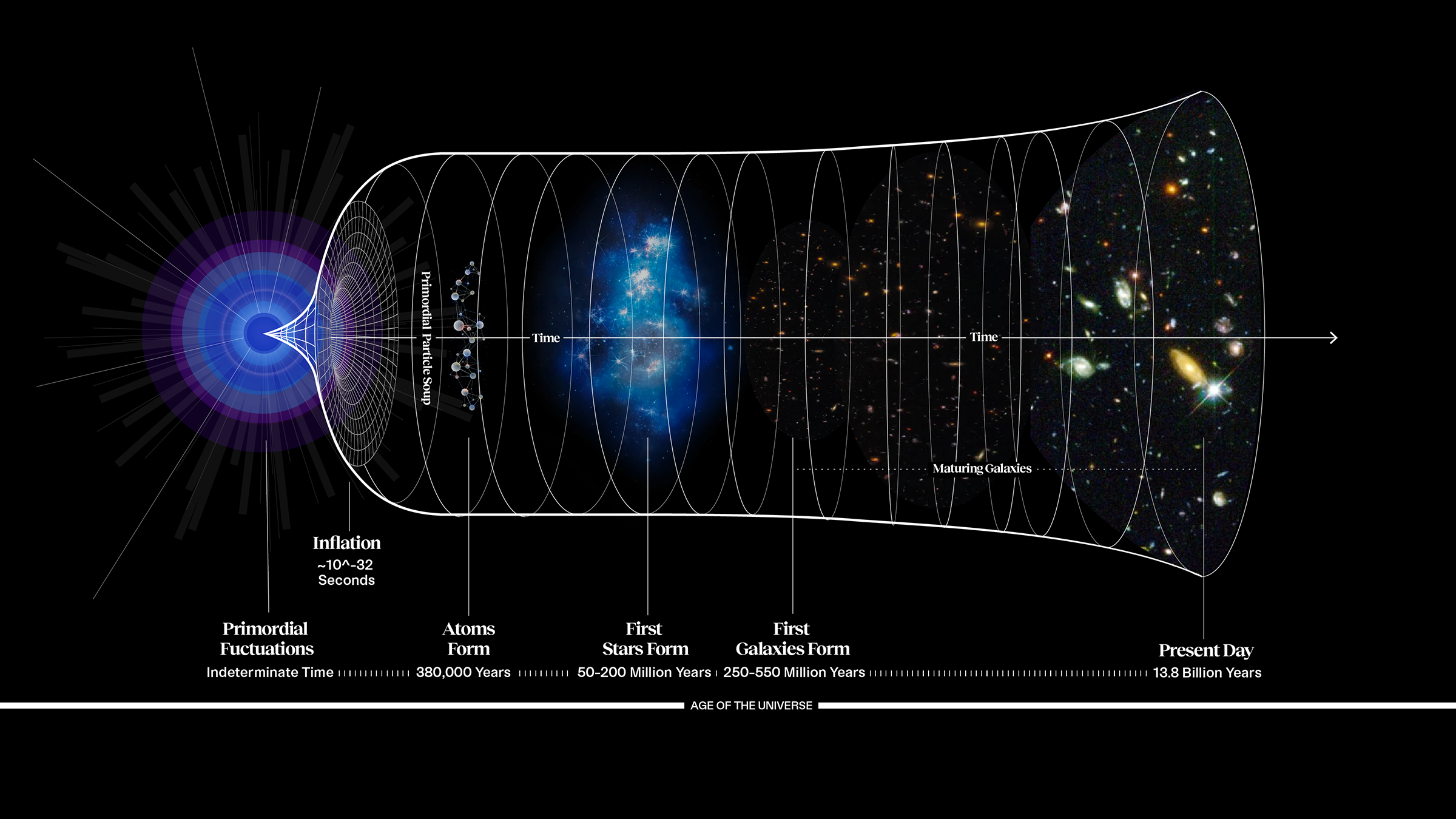
- For countless millennia, human beings and our ancestors wondered at the mysteries of the Universe: what it was, where it came from, and how it got to be the way it is today.
- Scientific advances, particularly during the 20th and 21st century, have now answered those questions: our Universe began with a hot Big Bang some 13.8 billion years ago, and that “bang” was preceded beforehand by cosmic inflation.
- But what was inflation like? How did it occur, and what type of inflation truly gave rise to our Universe? While many enormous questions have already been answered, several important ones still remain.
Imagine what it must have been like, as it was for so long throughout human history and prehistory, to look up at the wonders of the night sky in ignorance: not knowing what you were seeing or where any of it came from. All you could behold with your eyes were those glittering points of light in the sky: the Moon, the planets, the stars, a few deep-sky objects (or nebulae), and the tapestry of the Milky Way, with no way of knowing what they were made of, where they came from, or what any of it meant.
Today, the story is very different. Nearly all of the night sky objects we can see with our naked eye are objects present within the Milky Way galaxy. A few of those deep-sky objects turn out to be galaxies, with trillions of more galaxies — including small, faint, and ultra-distant ones — observable with superior tools. These galaxies all expand away from one another, with more distant objects expanding at greater speeds than nearer ones.
The expanding Universe swiftly led to the idea of the Big Bang, which was then confirmed and validated. The Big Bang was then modified to include an even earlier stage known as cosmic inflation, which preceded and set up the Big Bang’s initial conditions. That’s the current status of our understanding of the beginning as of today, in early 2024. Here are the biggest questions, both answered and unanswered, that we still have about the earliest phases of our Universe.
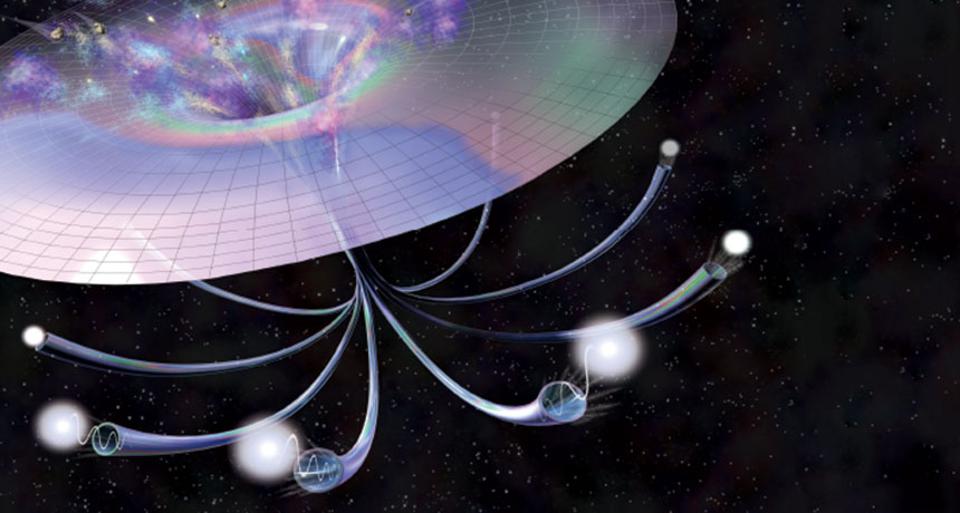
The inflationary hot Big Bang
Most of us have heard of the Big Bang: the notion that the Universe began from a very hot, very dense, and very uniform state, and then expanded, cooled, and gravitated, eventually giving rise to:
- protons and neutrons,
- atomic nuclei,
- neutral atoms,
- stars,
- galaxies,
- and a vast cosmic web of structure,
where within individual galaxies, things like heavy elements, rocky planets, and even life can eventually form. However, the Big Bang couldn’t have been the very beginning of the story, as a number of physical puzzles simply go unexplained if we insist on it.
- Why does the leftover glow from the Big Bang, the CMB (or cosmic microwave background), have the same properties (e.g., temperature) in all directions, especially if these distant, disconnected regions have never had time to exchange information with one another?
- Why does our Universe, where the combination of energy density and the expansion rate determines its curvature, appear perfectly spatially flat, rather than either positively or negatively curved?
- And why are there no leftover high-energy relics from this supposedly “arbitrarily hot” phase that the Universe would have achieved, early on, if the hot Big Bang truly represented the beginning of everything?

One possibility, famously noted by Alan Guth in the late 1970s/early 1980s (although others, such as Robert Brout, Alexei Starobinsky, plus Rocky Kolb and Stephen Wolfram, had similar ideas), is that the Big Bang wasn’t the beginning, but was preceded by a state of exponentially expanding empty space, which precedes and sets up the hot Big Bang. Further details were worked out by many, including Guth himself, Andrei Linde, Paul Steinhardt and Andreas Albrecht, as well as several others, concluding the following.
In an inflationary Universe, space was filled with a type of energy inherent to itself — perhaps some type of field energy, similar to today’s dark energy — causing it to expand not just rapidly, but relentlessly, and without bound. When inflation comes to an end, all (or at least, most) of that energy gets converted into particles and antiparticles, initiating the phase of the Universe we identify with the hot Big Bang. But now, because of inflation:
- Different regions all have the same temperature and density, because they all emerged from the same inflationary state.
- The Universe that emerges appears spatially flat, because the inflationary process stretched it so that it would be indistinguishable from a perfectly flat appearance, just as your own backyard appears “flat” on the surface of the Earth.
- And there are no leftover high-energy relics because any pre-existing ones were inflated away, and the maximum temperature that the Universe achieves when the hot Big Bang begins is now insufficient to create them again.
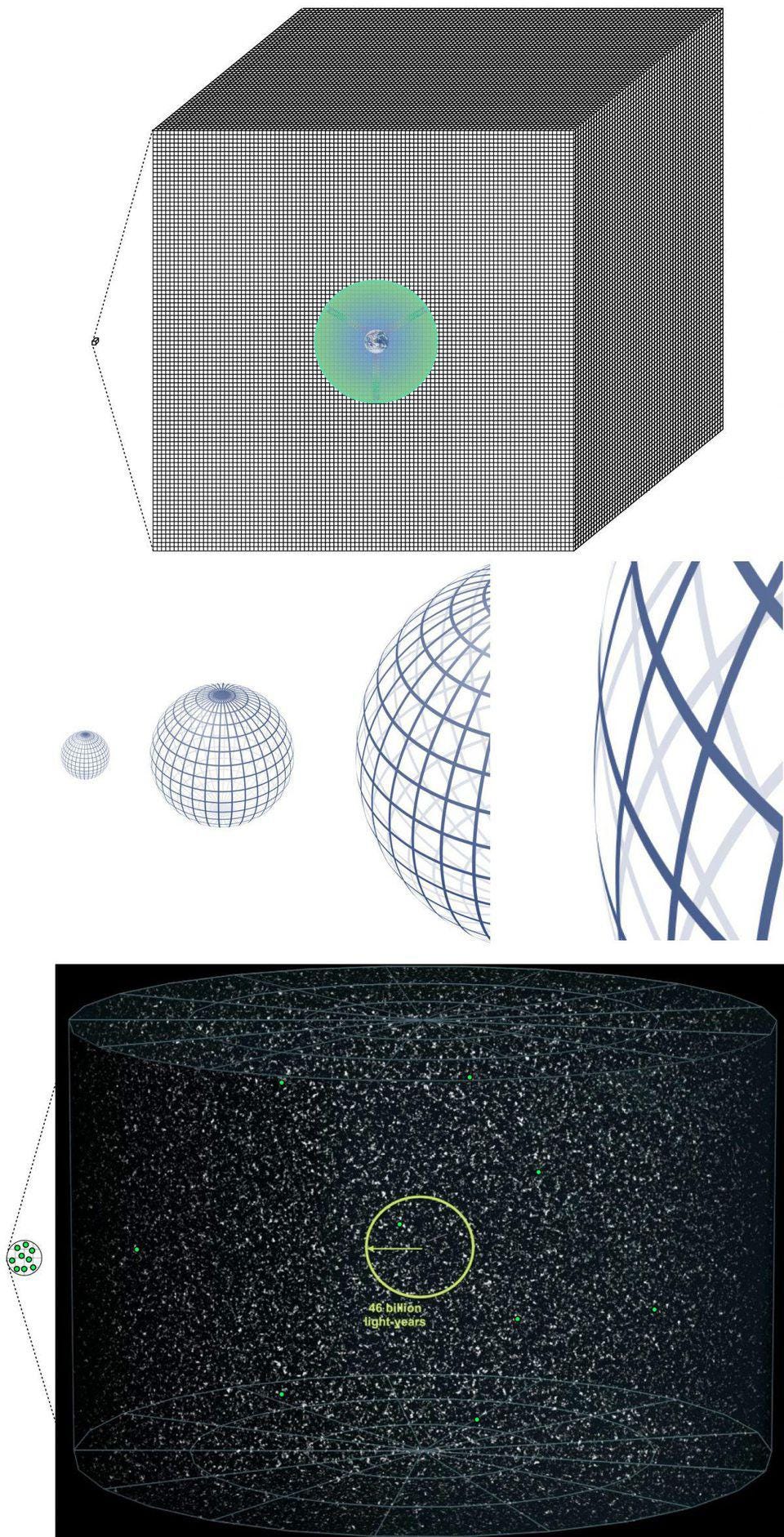
In other words, inflation not only reproduces the Big Bang’s successes, but it solves all three of the major physical puzzles that plagued the Big Bang beforehand. This lovely story was off to a compelling start, explaining the problems of uniform temperature, observed flatness, and the lack of high-energy relics that the standard hot Big Bang scenario struggled with. But in order to supersede an older scientific theory, a new one needs to make novel predictions that differ from the theory that preceded it.
For inflation, the simplest way to visualize it is as a field: where you start out as a ball on the top of some hill and roll down it. As long as you’re high on the hill, your Universe inflates; when you roll all the way down the hill and into a valley below, inflation ends and the energy from it gets converted into quanta, beginning the hot Big Bang. However, because all fields in nature ought to be inherently quantum, this leads to two types of quantum processes:
- quantum fluctuations that get stretched across the Universe and become density/temperature fluctuations, known as scalar fluctuations,
- and quantum fluctuations that generate a primordial spectrum of gravitational waves, known as tensor fluctuations.
Only the first class of these fluctuations has been detected today, but both types lead to a series of predictions, which allow us to test and constrain various inflationary scenarios and their relevance for our Universe.

Picturing quantum fluctuations
Try to imagine the Universe as it was during this inflationary state. Space swiftly becomes empty — completely empty — as any two nearby points are inexorably driven apart in an exponential fashion: where the distance between them doubles after a certain amount of time, then quadruples after twice that time, then octuples after three times that time, and so on. By the time that 1000 times that initial time interval have elapsed, even two points that were separated by the minimum physical distance that makes sense (the Planck length), initially, will now be farther apart than opposite ends of today’s observable Universe.
And yet, as this inflationary expansion occurs, there are still quantum fluctuations occurring on all scales throughout all of space. The fluctuations that occur on the smallest of scales get stretched to larger scales, while as the next moment elapses, new fluctuations appear on the smallest scales. As “older” fluctuations get stretched to larger scales, they are joined by “newer” fluctuations on smaller scales, with each set of fluctuations getting superimposed atop the older, now larger-scale fluctuations. Only when inflation comes to an end do these quantum fluctuations — both scalar-type and tensor-type — get converted into density fluctuations (for scalar) and gravitational wave fluctuations (for tensor) that seed the Universe when the hot Big Bang first takes place.
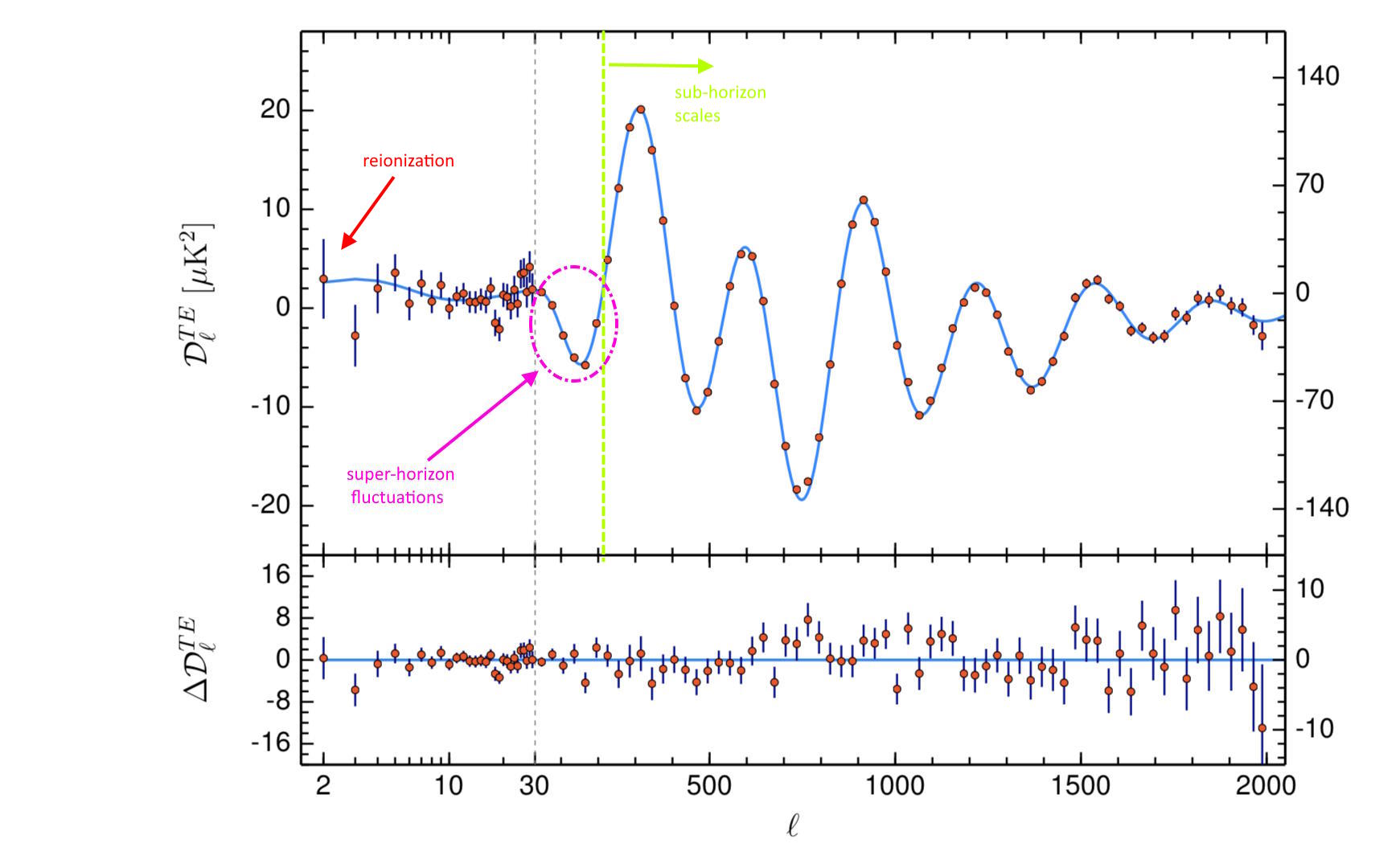
Inflationary successes
This leads to a number of predictions for how these fluctuations should impact the Universe we observe today, and those predictions either differ from those of the non-inflationary hot Big Bang or make concrete predictions whereas the hot Big Bang makes none at all. For some of these predictions, the data has already come in, and agrees wildly with what inflation leads us to expect will occur.
#1: Super-horizon fluctuations. There’s a limit to the size that any structure in the Universe can take on: the size set by the speed of light, the rate of cosmic expansion, and the amount of time that’s elapsed since the Universe’s inception. If there were no period of cosmic inflation, the upper limit to that size would be set by those factors, and is known as the classical horizon. If we observe coherent structures in the Universe that are larger than that size, e.g., super-horizon fluctuations, then we’ve found evidence for cosmic inflation. This was first measured in polarization data by WMAP, and has since been confirmed and measured to even better precision by Planck.
#2: Almost, but not perfectly, scale-invariant fluctuations. During most of cosmic inflation, the fluctuations that get stretched to larger scales have exactly the same properties as the new ones that appear later on, on smaller scales. However, there’s a small departure that happens from this at a critical moment in time: during the very final moments of cosmic inflation, just before it ends and gives rise to a hot Big Bang.
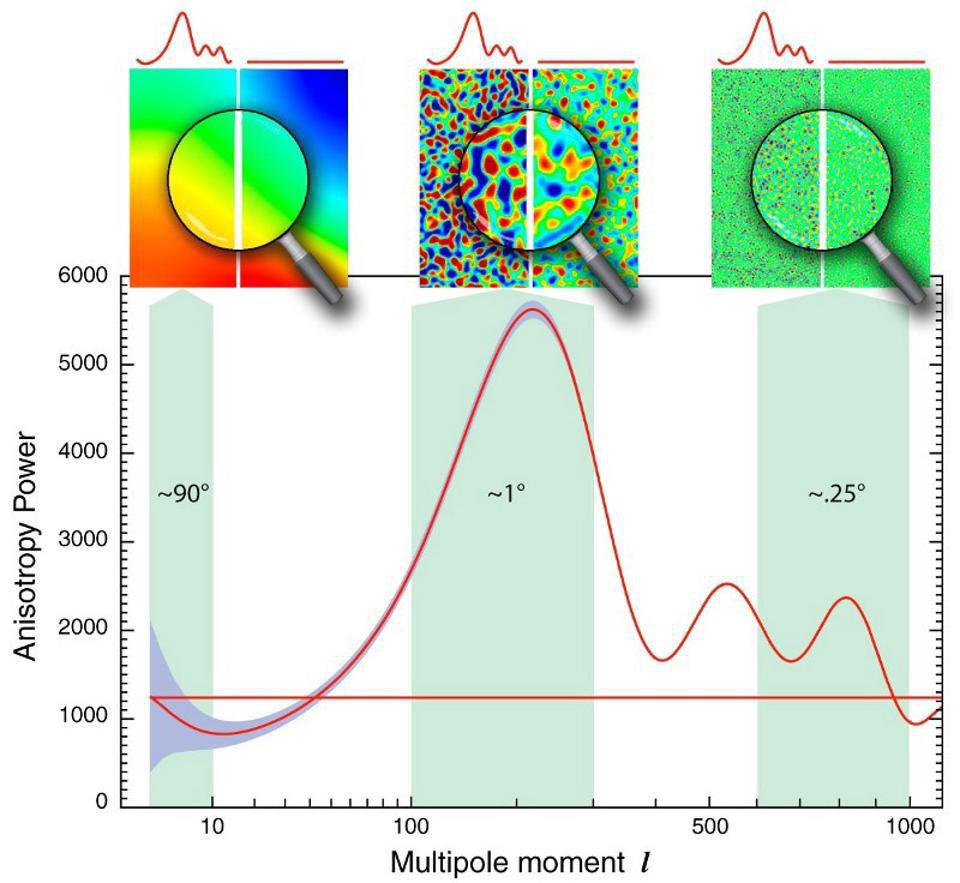
That critical set of moments corresponds to the smallest cosmic scales in the Universe today, while the earlier moments correspond to larger cosmic scales. We normally characterize this spectrum of fluctuations by a parameter called ns, or the scalar spectral index. If the fluctuations were perfectly scale invariant, then ns would equal 1 exactly, but we observe it to be just slightly smaller than 1: ns = 0.97. This is a spectacular confirmation of inflation, and perhaps the best way we have of constraining the types of inflation that could have occurred.
We also predict that:
- #3: the Universe is spatially flat to at least 1 part in a few tens of thousands,
- #4: the density fluctuations that arise will be 100% adiabatic (of constant entropy) in nature, as opposed to isocurvature (of constant spatial curvature) in nature,
- #5: with Gaussian statistics to their distribution, meaning that the temperature fluctuations observable in the CMB will follow a Bell curve (normal distribution) on all angular scales.
To the best of our observations, these predictions have all been confirmed. The Universe is spatially flat to about 1 part in 400 (the best we’ve ever measured it), the density fluctuations are at least 98.3% adiabatic and at most 1.7% isocurvature (the best we’ve ever measured it), and the fluctuations obey Gaussian statistics as far as we can tell, with no detectable non-Gaussianity showing up to date.
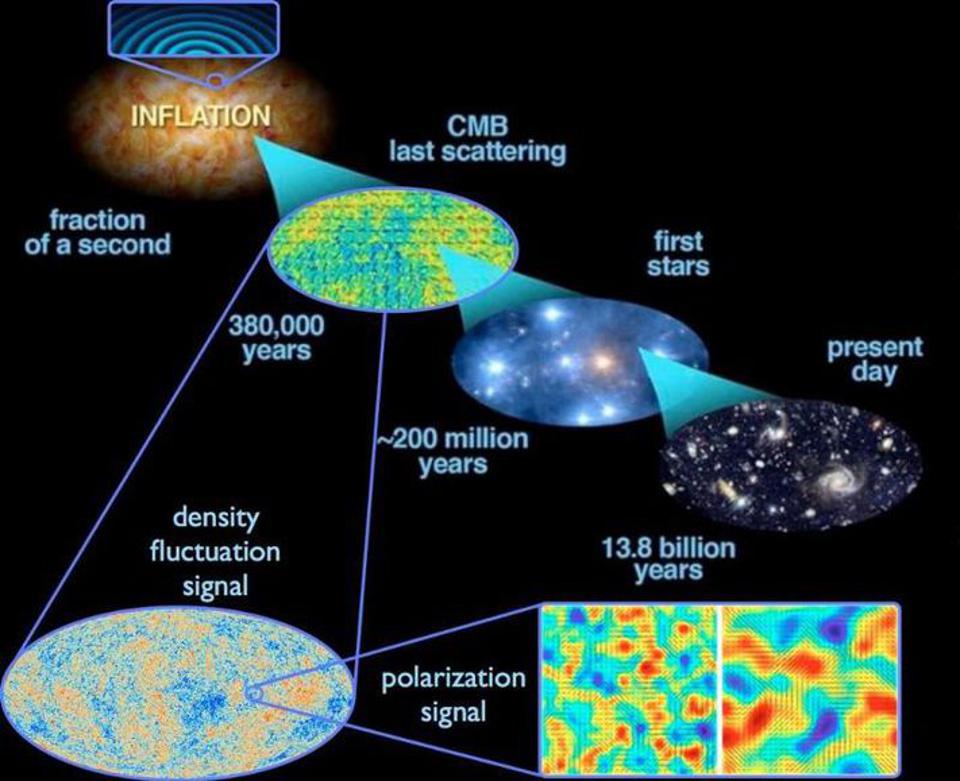
Inflation’s unanswered questions
This seems like a remarkable success story for cosmic inflation, and in many ways, it truly is. 50 years ago, we had cemented the hot Big Bang as accurately describing the early phases of our Universe, but it failed at explaining a set of conditions that must have existed back then, and contained numerous pathologies (or puzzles) that had no solution. When cosmic inflation came along, it was recognized that it could resolve these problems, but that new, testable predictions needed to be extracted from them.
We have now entered a golden era for cosmology, where next-generation experiments that probe the fluctuations and polarization of light imprinted in the cosmic microwave background (CMB) are being designed and constructed. We have confirmed a number of predictions that inflation makes, ruling out the hot Big Bang without inflation and also ruling out a number of inflationary models that fail to match the data.
But if we had better data, we could imagine even more stringent tests of inflation that could:
- confirm its predictions still further,
- teach us which models agree with the data and which are ruled out,
- or could surprise us, and show us that certain predictions are not, in fact, borne out by nature.
Although we do not have the data yet, here are 5 unanswered questions about inflation that may still be answered with superior future data.
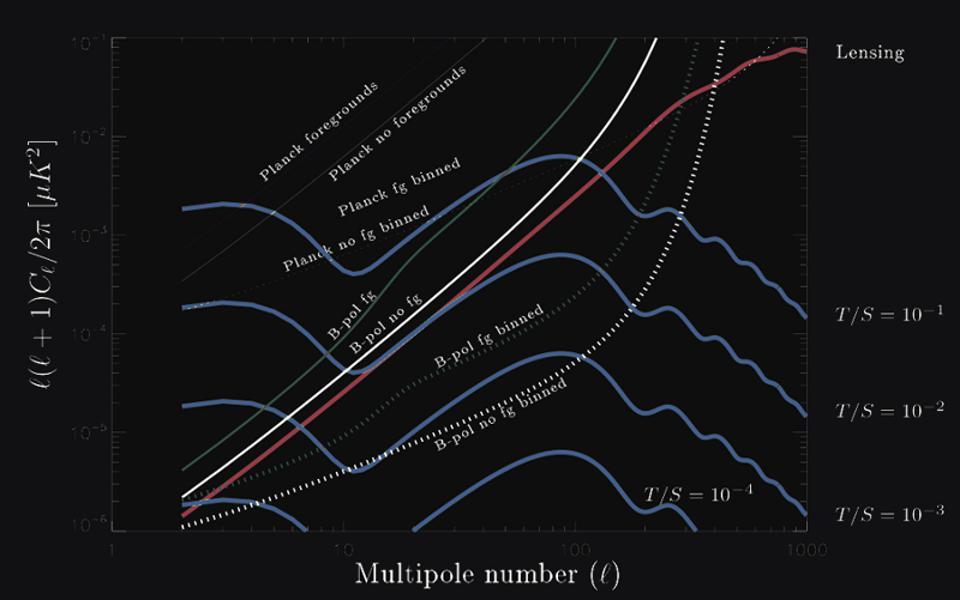
#1: Are there tensor fluctuations, or primordial gravitational waves, present within our Universe? The graph above is remarkable: it shows the spectrum of gravitational waves predicted to be generated by inflation. The only problem? The spectrum is easy to determine, but the amplitude of the spectrum is highly model-dependent. If the tensor spectrum has a large amplitude, then the ratio of the tensor spectral index (nt) to the scalar spectral index (ns) will be large, and we’ll be able to observe it. Right now, our best constraints on that ratio tell us that it’s less than 0.036, as determined by the Bicep-Keck collaboration.
#2: Does the scalar spectral index, ns, have a constant value, or does it change with scale (i.e., “run”) as predicted by inflationary models? Just as a ball rolling down a hill can change its acceleration if the slope of the hill changes, the expectation is that the scalar spectral index, ns, will “run” by a small amount: around 0.1%, according to most inflationary models. Will we be able to measure this running, and if so, will it be consistent with inflation’s predictions, or will it be either too large or too small?
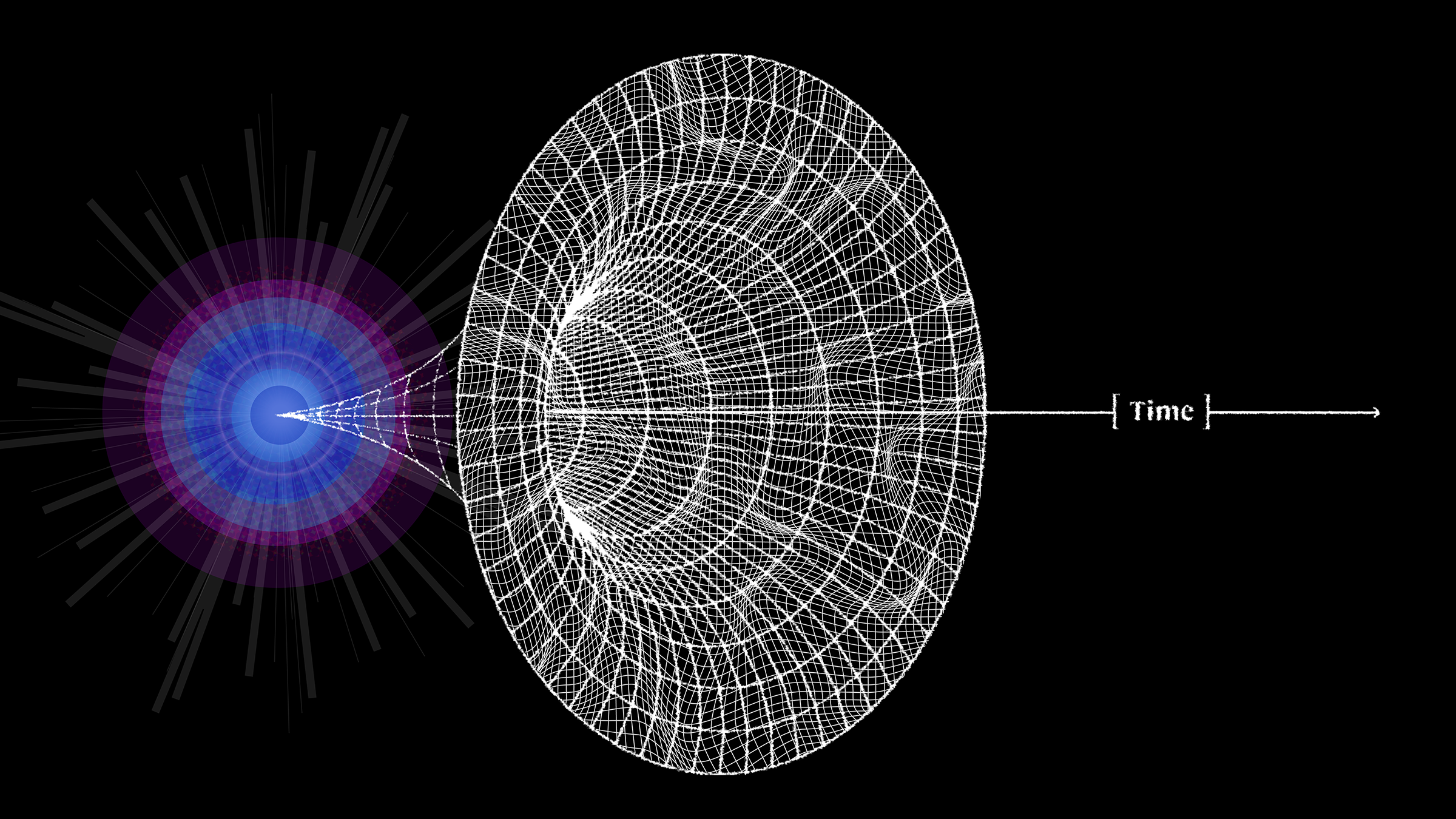
#3: Is the Universe’s geometry exactly flat, or, as inflation predicts, are there tiny departures from perfect flatness? Although inflation stretches the fabric of the Universe to be indistinguishable from flat, the quantum fluctuations imprinted during inflation can also imprint a non-zero amount of spatial curvature to it. According to various inflationary models, the amount of that curvature can vary from 1 part in 10,000 down to 1 part in 1,000,000. If there’s more or less curvature than that, it could spell trouble for inflation, while measuring curvature in precisely that range would be another spectacular confirmation for inflation.
#4: Are there any scalar fluctuations that exhibit any amount of non-Gaussianity to their statistics? Again, we do expect that if we get all the way down into the weeds, eventually we’ll find a small, non-zero departure from a perfect Bell curve to the temperature fluctuations that we see. Will the amount of non-Gaussianity agree with inflation’s predictions, or will it be either too small or too large?
#5: And finally, are there any resonant features in the spectrum of scalar fluctuations? We expect the answer will be “no,” as inflation predicts, but you have to look for the unexpected if you want to give nature a chance to surprise you.
Making measurements sensitive enough to test these five hitherto untested predictions is an ambitious goal, but when it comes to a question as important as “where our Universe came from,” not even making the attempt to find the answer may be the greatest folly of all.