How charges and masses create the Universe around us
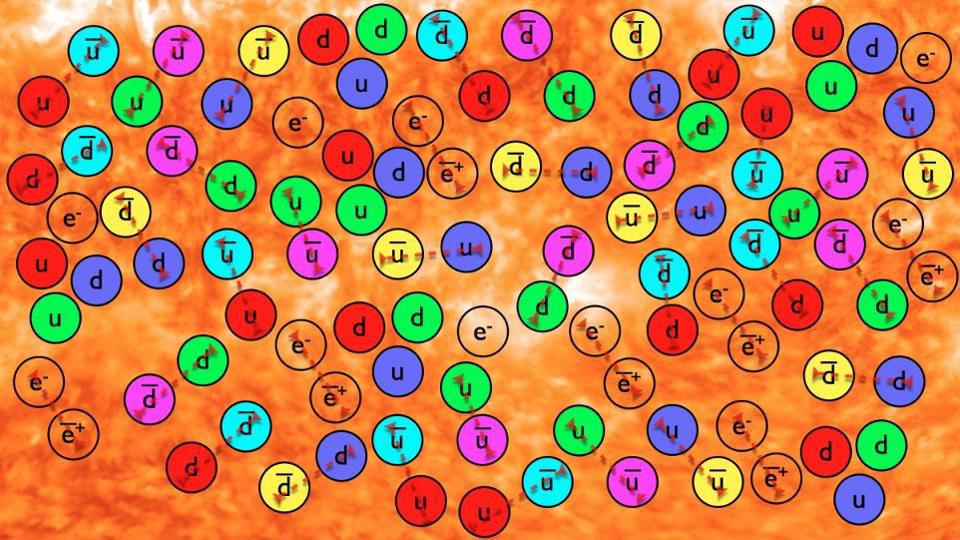
- It’s hard to believe, but the incredibly rich, varied world and Universe we see around us is composed, at its core, of just a few different species of fundamental particles.
- Quarks bind together to make protons and neutrons, which make nuclei, which (with electrons) make atoms, which link together to make molecules, planets, stars, galaxies, and more.
- What is the reason that all of these structures exist with the properties they possess today? At a fundamental level, it’s dictated by two simple things: mass and charge. Here’s how.
When we examine everything that exists, from atoms and molecules to macroscopic objects to planets and stars and even grander structures, we run into a fascinating puzzle: the fact that everything we see, observe, and know of is made from the same small set of fundamental particles. There are only a few dozen particles and antiparticles that exist as part of the Standard Model, along with the still-mysterious dark matter and dark energy. As simple as the Standard Model itself is, there are only a few particles that are required to understand almost everything:
- the up-and-down quarks, which make up protons and neutrons,
- the gluons, which hold protons, neutrons, and all atomic nuclei together,
- the electrons, which bind with atomic nuclei to make neutral atoms,
- the photon, which is the particle that mediates the electromagnetic force and is the quantum associated with light,
- and the neutrino (and its antimatter counterpart, the antineutrino), playing a major role in the weak nuclear interaction but otherwise of relative unimportance, cosmically.
Along with dark matter and dark energy, these simple species of fundamental particles are primarily responsible for creating the Universe we recognize, along with everything in it. Perhaps even more profoundly, just two of the properties of these fundamental particles, charges and masses, are ultimately responsible for the enormous variety of structures that can exist within our Universe, as the laws of physics work in the same, consistent fashion every time when it comes to each combination of these fundamental particles. Just charge and mass, in all their permutations, give rise to the entirety of the known Universe. Here’s how.
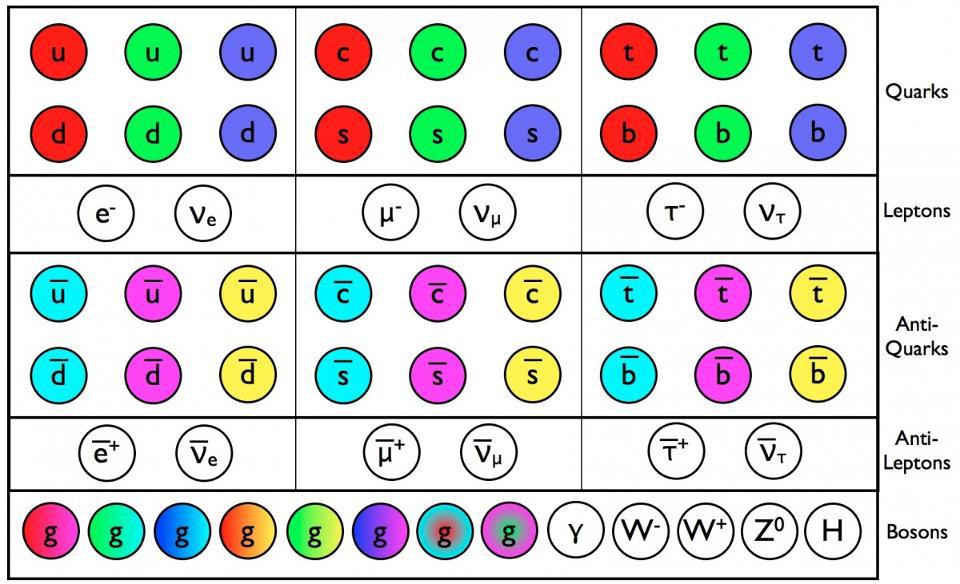
Above, you can see a charge of the various particles of the Standard Model. There are the fermions: the six flavors of quark and their corresponding antiquarks, the three species of charged lepton (electron, muon, and tau) as well as their antiparticle counterparts, along with the three types of neutrino and antineutrino. There are also the bosons: the eight gluons, the three heavy weak bosons (W and Z particles), the photon, and the Higgs boson. The quarks, charged leptons, W and Z particles, and the Higgs boson all have substantial masses, while the neutrinos and antineutrinos only have tiny masses and the gluons and photons have no rest mass at all.
However, what truly separates these different species of particle from one another goes way beyond their masses and whether they’re fermions (with half-integer spin) or bosons (with integer spin). There’s also how these particles (and antiparticles) are charged, which determines which forces they interact with.
- The quarks and gluons have color charge inherent to them, enabling them to experience the strong nuclear force, which is mediated by the gluons.
- The quarks and charged leptons have electric charge inherent to them (as do the W and Z bosons), enabling them to interact electromagnetically, where the electromagnetic force is mediated by the photon.
- And there are also weak isospin and weak hypercharge, experienced by all quarks and leptons (i.e., all of the fermions), which enable interactions through the weak nuclear force, mediated by the W and Z bosons.
Those three types of interaction, when you combine them with gravity (which is most famous for interacting with massive particles, but which affects massless ones as well), empower the particles of the Standard Model to assemble together to create the Universe we recognize.
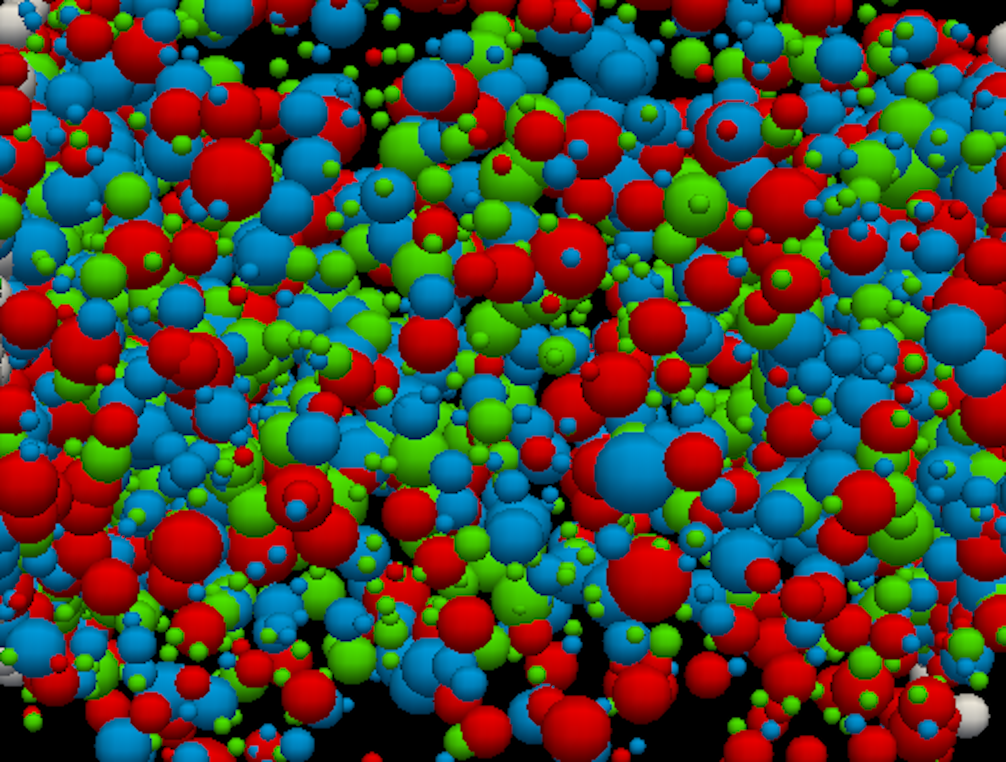
Although our Universe followed a series of cosmic steps to create the structures within it, we don’t need the hot Big Bang to recur in order to understand them in a laboratory setting. Simply beginning with the necessary particles (or, in the case of composite particles, their constituents) under the right energy and density conditions will inevitably result in the ultimate creation of bound, heavy structures that are stable or quasi-stable, even at modern-day low energies. Early on in cosmic history, everything was so hot and dense that we didn’t have protons, neutrons, or any other form of hadron; we only had free quarks (and antiquarks) and gluons, present in a hot, dense state of matter known as a quark-gluon plasma.
After only a brief fraction-of-a-second elapses, those quarks and gluons wind up bound together into protons and neutrons: very massive composite particles compared to the light quarks and massless gluons that comprise them. Even though the quarks have electric charges, and even though the like-charged quarks electrically repel one another, they also have a color charge to them. That color charge leads to a force between the quarks — mediated by gluons — that’s spring-like in nature: it goes to zero at very short distances, like an unstretched spring, but becomes large and attractive (and, importantly, more powerful than even the electromagnetic force) at greater distances.
The energy from the strong interactions, bound up in the gluon fields, is tremendous: it’s responsible for ~99% of the rest mass (via E = mc²) of the proton and neutron, while the distance scale of the electromagnetic and strong interactions, combined, leads to the physical size of baryons like protons and neutrons of just under 1 femtometer (10-15 meters).

Then, protons and neutrons — initially free particles in the early Universe, upon their creation — can be fused together: either in the hot, dense conditions that were present during the hot Big Bang, or later on in the interiors of sufficiently massive, hot stars. Although protons and neutrons:
- are massive,
- are either positively charged (protons) or electrically neutral,
- and have no net color charge (being composed of three colored quarks, red + green + blue, which equals “white” or “colorless” when combined),
they’re still composite entities that are fundamentally composed of quarks and gluons. Those quarks in particular, although they have their own masses and electric charges, also have a color charge to them, individually. Even though protons and neutrons, as a whole, don’t possess a net color charge, their color charged constituents (the quarks) can interact not only with one another, but with other color charged particles (i.e., other quarks) that are in close-enough proximity to them.
This leads to a phenomenon commonly called “the residual strong interaction,” where instead of modeling the strong nuclear force between individual quarks by the exchange of gluons, we model the strong nuclear force between individual baryons (e.g., protons and neutrons) by the exchange of mesons: quark-antiquark pairs. This residual force drops off very quickly with distance, becoming negligible at the scale of only a few femtometers. But it’s strong enough that it allows multiple protons (along with neutrons) to successfully bind together in a stable or quasi-stable configuration, sufficiently strong to overcome the electrostatic repulsion between like-charged particles (e.g., protons). Because of the residual strong force’s very short range, elements heavier than lead-208 are all inherently unstable, but a wide variety of stable atomic nuclei — elements and isotopes — can form with multiple protons and/or neutrons all bound together.

Once you have atomic nuclei, which always have a net positive electric charge, you have to recognize that in order to form more complex structures, you need something further to rescue you from electrostatic repulsion. The strong nuclear force can do it, but only on small (< ~10-14 meter) scales: scales of an atomic nucleus, but no larger. How, then, can we hope to form larger, more complicated structures within our Universe?
By introducing an equal number of negative electric charges into the Universe: for every proton, there needs to be a corresponding electron. Although electrons are similar-but-opposite to protons in terms of electric charge, with the same magnitude charge but the opposite sign, they also have an important difference from protons: they’re much, much lighter. Whereas one electron is sufficient to balance out the electric charge from a proton, it would take over a thousand electrons, 1836 electrons to be precise, to match the mass of just one proton.
It’s for that reason — equal and opposite charges but vastly different masses — that when neutral atoms form, they’re enormous compared to the sizes of the individual particles that compose them: a whopping ~1 Angstrom (10-10 meters) in size. Unlike atomic nuclei, which require extreme temperatures and energies to either fuse together or blast apart, atoms can have electrons bound or unbound from them fairly easily: requiring only about a millionth of the energy a nuclear reaction does to proceed.

What can you do, then, once you have multiple atoms, including atoms of different species, where electrons are bound to protons and other atomic nuclei in electrically neutral configurations?
The answer is simple, straightforward, and profound: atoms can link up to form more complex structures: molecules, enzymes, salts, and more. Many of these compounds will form spontaneously, simply by bringing the necessary component atoms into contact with one another, while others require some sort of chemical reaction, often requiring energy or some other catalyst (including a chemical catalyst) to create them.
Unlike atoms, which are limited in size to around ~1 Angstrom, molecules can be enormous, ranging from the scale of nanometers (10-9 meters) up to even macroscopic scales, as DNA, crystalline structures, and carbon nanotubes can all be seen with the unaided human eye. When it comes to molecules, it’s the electromagnetic forces between the component atoms — and particularly between the electrons within those atoms — that enable molecular assembly and maintain molecular stability. Once again, electric charge dominates these scales, and is responsible for the entities that form on these scales.

However, even though each individual atom has an extremely small mass, even grander structures can form if you assemble enough of them together. At a fundamental level, gravity is by far the weakest of the known fundamental forces. If you take two protons and have them touch each other, so that their centers are separated by just a little over 1 femtometer, you’d find that:
- electrostatic repulsion was the dominant force,
- followed closely by the strong nuclear force, still more than 10% as strong as the electromagnetic force,
- followed more distantly by the weak nuclear force, which is about a factor of a million weaker,
- followed extremely distantly by the gravitational force: a force that’s about a factor of ~1030 still weaker than the weak nuclear force.
But if you take a large number of atoms all together — a number much, much greater than a mere ~1030 of them — then the gravitational force is going to dominate. With neutral color charges and neutral electric charges, overall, it’s the cumulative rest mass of all the particles in your system, the neutral atoms, that are eventually going to win. Get enough of that massive stuff together, and not only will it gravitationally bind itself together, but because of the electromagnetic interaction radiating heat and angular momentum away, it will enable that massive stuff to gravitationally collapse.
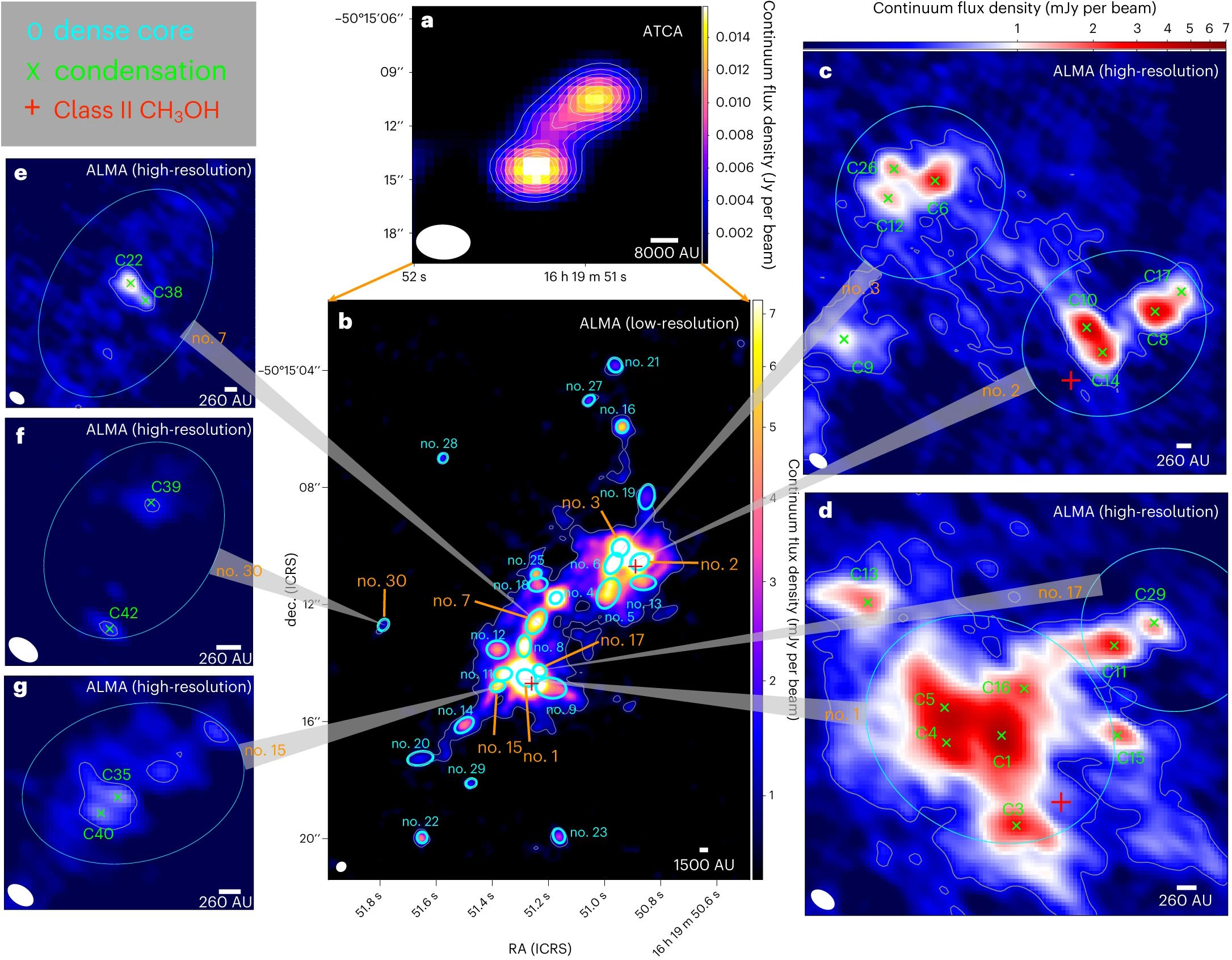
When it comes to collections of atoms, the key to making large structures — i.e., structures larger than mere molecules — is to first accumulate a cloud of gas so massive that it does begin to collapse under its own gravity. Because the cloud is made of atoms, they’ll:
- collide,
- stick together, shedding energy,
- heat up,
- radiate that heat away (i.e., cool),
- and occupy progressively smaller volumes,
which leads to both collapse and fragmentation. Where the densities get the greatest the fastest, and where the largest amounts of mass accumulate the most rapidly, new stars will form: marked by the initiation of nuclear fusion at sufficient temperatures and densities in their cores.
And this is hugely important. Whereas planets, moons, asteroids, and other small bodies can gather together in a collapsed form, stars have something else going on: nuclear fusion occurring inside of their cores. Gravitation, remember, is an inward, attractive force; it takes the structure of atoms themselves to hold those objects up against gravitational collapse. With stars, however, nuclear reactions occur inside of them, converting mass into energy and radiating that energy outward as photons: radiation, which exerts an outward pressure. This is why stars are large and puffy; that extra radiation holds them up against further gravitational collapse. Without it, their cores would contract or even collapse, while their outer layers would escape away: precisely what happens in the formation of stellar remnants like white dwarfs, neutron stars, and even black holes.

And finally, on the absolute largest of cosmic scales, we come to objects outside of our own Milky Way: galaxies, galaxy groups and clusters, cosmic filaments, large wall-like structures of galaxies, and on even grander scales, the enormous cosmic web. When it comes to these scales, gravitation isn’t just dominant, it is the only force that matters. The way it matters is different than what most people intuit, as whether a massive region can collapse to form a structure is dependent on which of two competing factors wins:
- the expansion of the Universe, which works to drive matter apart and rarify it,
- and gravitation, which mutually attracts matter particles to one another and fights against the expansion.
Based on the properties that we’ve measured about the Universe, it is no longer atoms or any of the Standard Model particles that dominate physics and astrophysics on these scales; it’s dark energy, which dominates the expansion, and dark matter, which dominates the effects of gravitation.
The fact that we have the relative energy densities we do (68% dark energy, 27% dark matter, 5% “normal” stuff), that the Universe is as old as it is (13.8 billion years since the start of the hot Big Bang), and the fact that all signals are limited by the speed of light (which is also the speed of gravity) dictate what massive structures will form on which scales. This determines the masses and distributions of galaxies, how they cluster together, how cosmic voids form and grow, and how there ought to be no cosmic structures, at present, larger than about 1.4 billion light-years in extent. This is congruent with what we observe, and although some have claimed the existence of larger structures, the best science that we have today leaves us confident that they don’t actually exist.

On every scale that we look at, it’s the charges and masses of the relevant objects involved that determine not only what forms within the Universe, but how large the structures are that result. The particles of the Standard Model may all be truly point-like, with no “minimum size” to them, but the bound structures that they form do have finite sizes.
- Protons and neutrons, made of quarks and gluons, are about a femtometer in size.
- Bound atomic nuclei, made of protons and neutrons, are a few femtometers, perhaps up to 10 or so, across.
- Atoms, composed of nuclei and electrons, are about an Angstrom in size.
- Molecules, composed of atoms all linked up together, range from about a nanometer to up to a meter or so in size.
- Bound collections of atoms that go beyond molecular structures range from dust grains up to stars, with stars being large, puffy, and diffuse owing to nuclear reactions that take place within them.
- And the largest structures of all, from galaxies up to the grand cosmic web, are shaped not by atoms at all, but by dark matter and dark energy.
Charges (including color charge and electric charge), masses, and the laws of physics are all that are required to explain the enormous, rich variety of structures that we encounter in our Universe today, while simultaneously explaining why structures aren’t larger or smaller than certain values.
But there’s a key thing to keep in mind: even though we know all of this and can weave a consistent story out of it, we still don’t know why nature has the values that it does. We don’t know why the charges have the strengths or signs that they do. We don’t know why particles and antiparticles have the masses that they do, or why some of them (neutrinos, looking at you) are massive or why some of them are so much more massive than others. If these properties were altered, even slightly, the Universe would be wildly, perhaps even catastrophically, different. Even with all that we know, there are still deep, fundamental puzzles just waiting to be solved.