How The Planck Satellite Forever Changed Our View Of The Universe
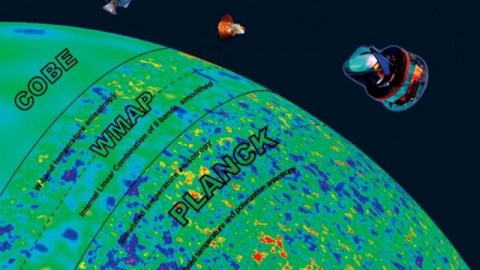
Humanity’s greatest-ever view of the Big Bang’s leftover glow has just released their final analysis. Here’s what we’ve learned.
It’s been more than 50 years since humanity discovered a uniform bath of low-energy, microwave radiation originating from all regions of the sky. It doesn’t come from the Earth, the Sun, or even the galaxy; it originates beyond every star or galaxy we’ve ever observed. While it’s discoverers didn’t know what it initially was, a group of nearby physicists were in the midst of designing an experiment to look for that exact signature: the theoretical leftover glow from the Big Bang.
Initially known as the primeval fireball, we now call in the cosmic microwave background (CMB), having measured its properties exquisitely. The most advanced observatory to ever measure its properties is the European Space Agency’s Planck satellite, launched in 2009. The satellite took its full suite of data over many years, and the scientists working on it have just completed and released their final analysis. Here’s how it’s changed our view of the Universe forever.
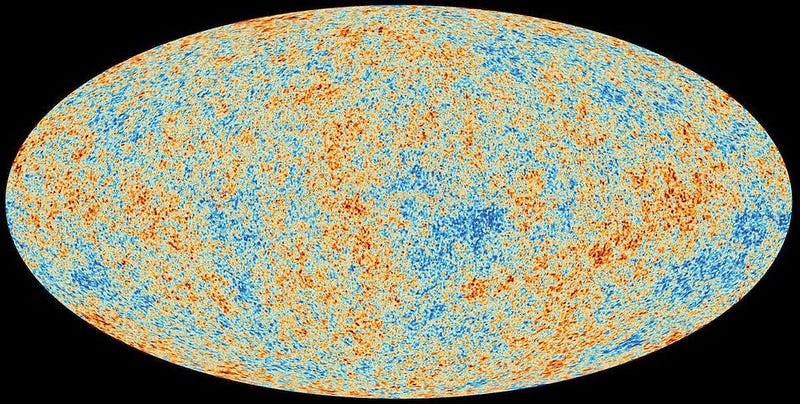
This baby picture of the Universe, whose light was emitted when the Universe was only 380,000 years old, is far more exquisite than any that came before. In the early 1990s, the COBE satellite gave us the first precision, all-sky map of the cosmic microwave background, down to a resolution of about 7 degrees. About a decade ago, WMAP managed to get that down to about half-a-degree resolution.
But Planck? Planck is so sensitive that the limits to what it can see aren’t set by instruments, which can measure down to 0.07° or so, but by the fundamental astrophysics of the Universe itself! In other words, it will be impossible to ever take better pictures of this stage of the Universe than Planck has already taken. Additional resolution will not get you better information about our cosmos.
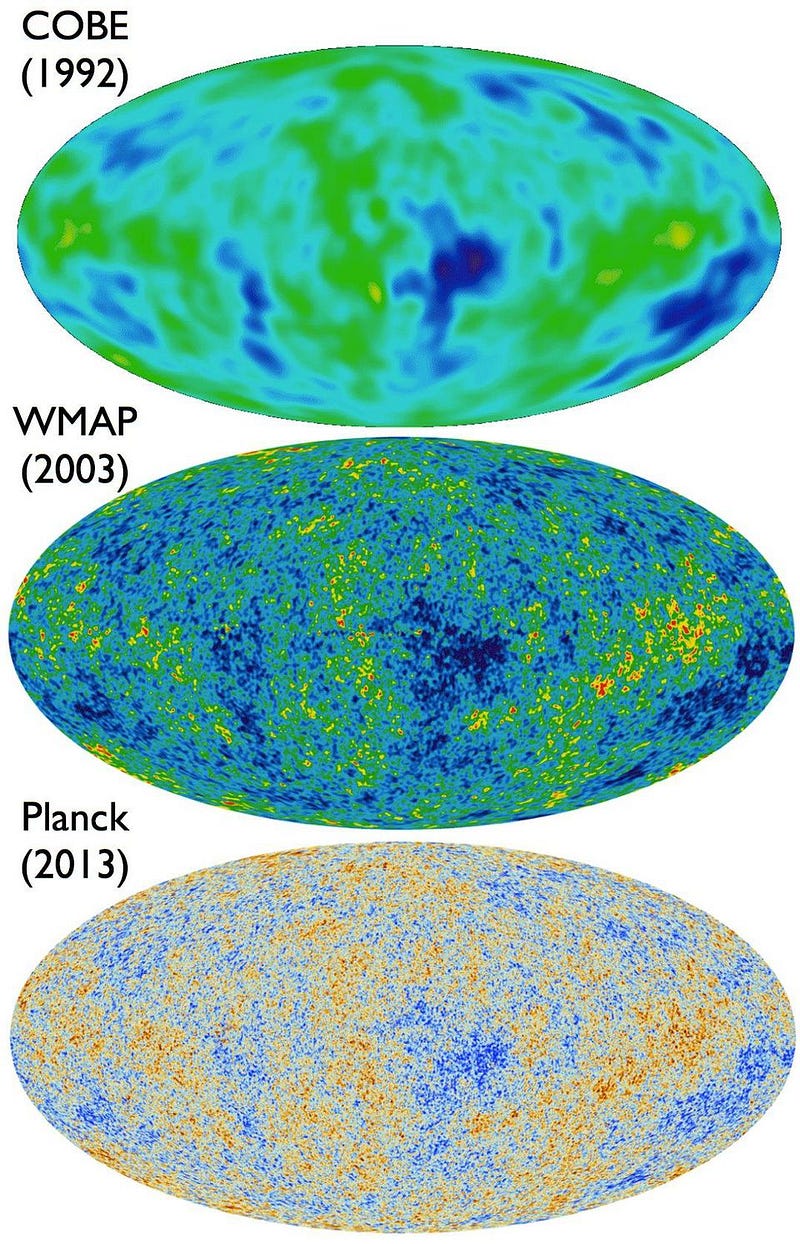
Planck has also measured this radiation and its fluctuations in more frequency bands (nine in total) than any satellite that came before. COBE had four (only three were useful), and WMAP had five. COBE could measure temperature fluctuations that were approximately 70 microkelvin (µK) in magnitude; Planck can get down to precisions of around ~5 µK or better.
The high resolution, the capabilities of measuring the polarization of this light, and the multiple frequency bands have enabled us to understand, measure, and subtract out the effects of dust in our galaxy better than ever before as well. If you want to understand the Big Bang’s leftover glow, you have to understand to equal-or-better precision the effects that could contaminate that signal. Before we extract any cosmological information, that step needed to happen.
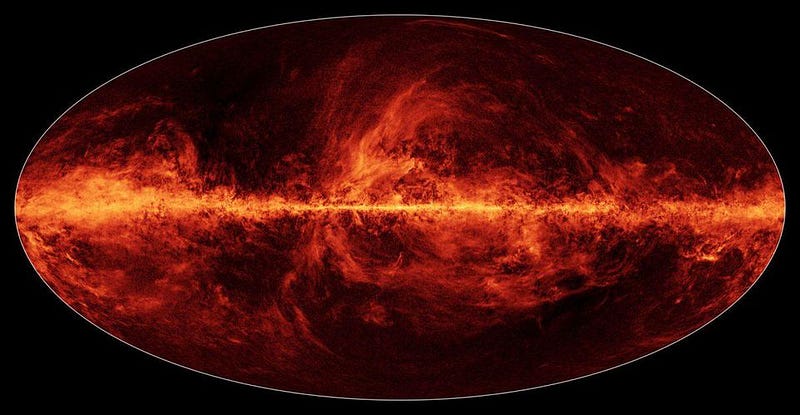
Once you have the full signal from the early Universe, you can then analyze it to extract all the information possible. This means using the temperature fluctuations on large, intermediate, and small scales to figure out things like:
- how much normal matter, dark matter, and dark energy are in the Universe,
- what the initial distribution and spectrum of density fluctuations were,
- and what the shape/curvature of the Universe is.
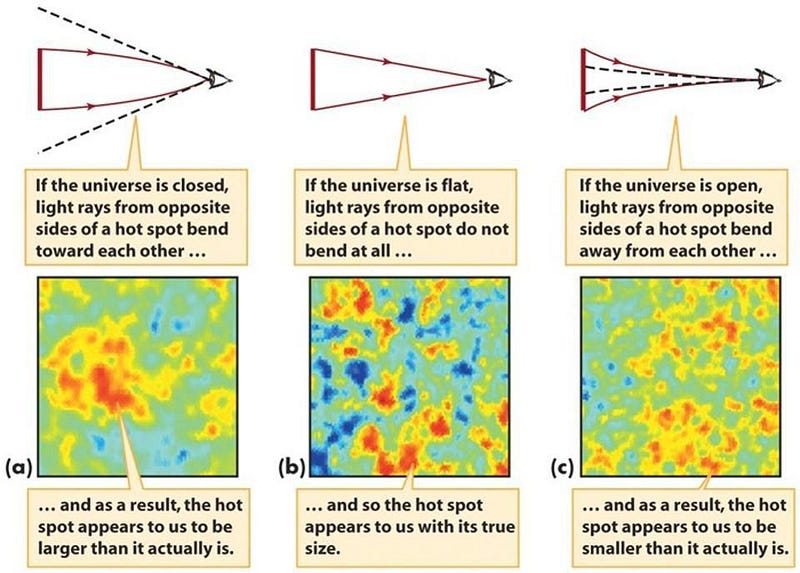
What happens on different scales are all independent of one another, but are highly dependent on what the Universe is made of. We can also look at a variety of polarization signatures of this radiation, and learn even more, such as:
- when the Universe became reionized (and, therefore, when star formation reached a certain threshold),
- whether there are fluctuations on scales larger than the horizon,
- whether we can see the effects of gravitational waves,
- what the number and temperature of neutrinos were back then,
and much more. While the temperature of the CMB is still 2.725 K, the same value it’s been known to have for decades, many other things have changed. With all that, here’s how our view of the Universe has forever been changed by Planck.
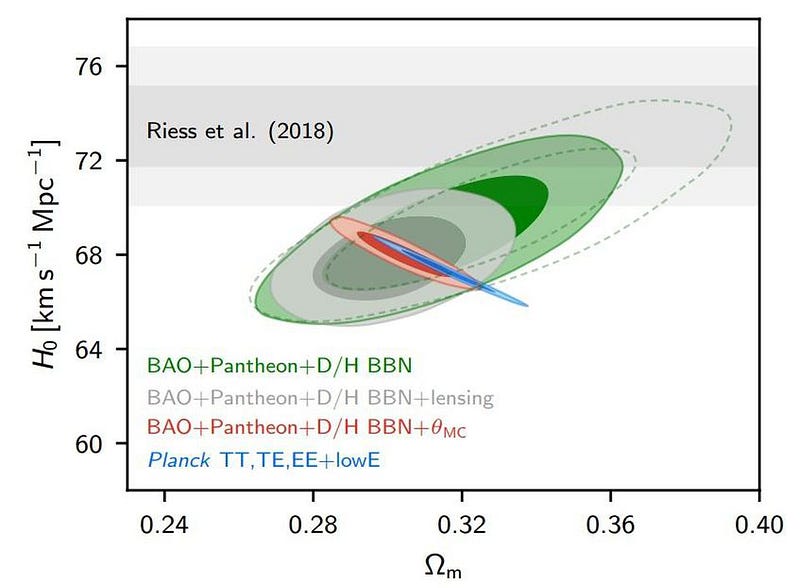
The Universe has more matter and is expanding more slowly than we previously thought. Before Planck, we thought the Universe was about 26% matter and 74% dark energy, with an expansion rate (in units of km/s/Mpc) in the low-70s.
Now?
The Universe is 31.5% matter (where 4.9% is normal matter and the rest is dark matter), 68.5% dark energy, with a Hubble expansion rate today of 67.4 km/s/Mpc. That latter figure has such small uncertainties on it (~1%) that it’s in tension with measurements from the cosmic distance ladder, which indicate a rate closer to 73 km/s/Mpc. This last point is probably the biggest remaining controversy surrounding our modern view of the Universe.
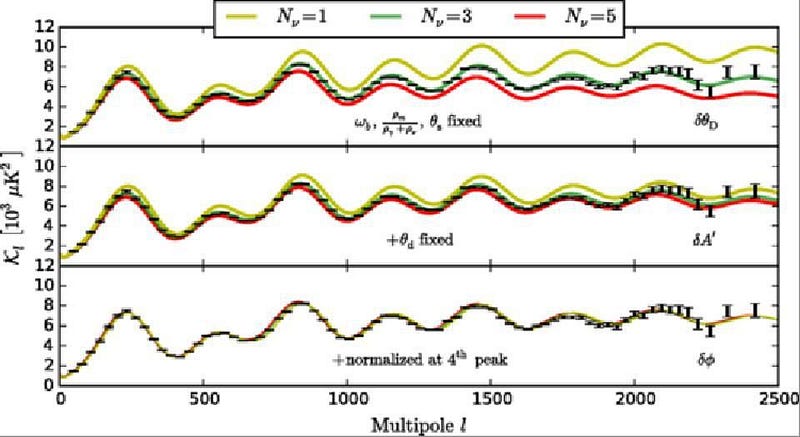
We know that there are only three types of neutrino from Planck, and that the mass of any individual neutrino species can be no more than 0.04 eV/c²: more than 10 million times less massive than an electron. We also know that these neutrinos had a cosmic temperature that would correspond to 72% of the temperature/kinetic energy that the CMB photons have; if they were massless, the temperature would be just 2 K today.
We also know that the Universe is really, really flat in terms of its overall spatial curvature. By combining the Planck data with the data from large-scale structure formation, we can state that the curvature of the Universe is no greater than 1-part-in-1000, indicating that the Universe is indistinguishable from perfectly flat.
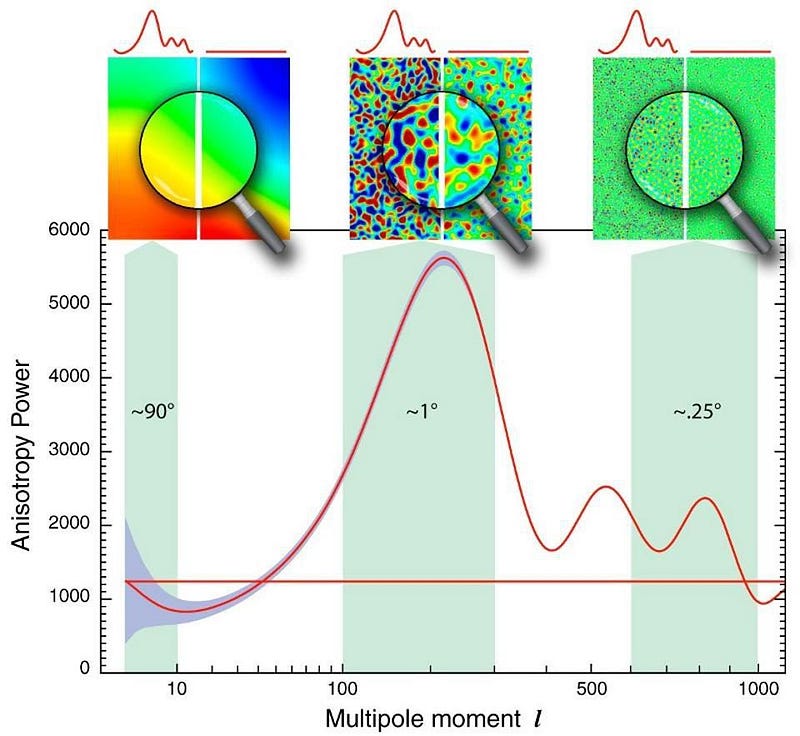
We also have the best-ever confirmation that the density fluctuations align perfectly with what the theory of cosmic inflation predicts. The simplest inflation models predict that the fluctuations the Universe was born with would be almost, but not quite, the same on all scales, with slightly larger fluctuations on large scales than small ones.
For Planck, this means one of the quantities it can derive, n_s, should be almost equal to 1, but just a little less. Planck’s measurements are the most precise ever, and confirm inflation’s spectacularly: n_s = 0.965, with an uncertainty of less than 0.05%.
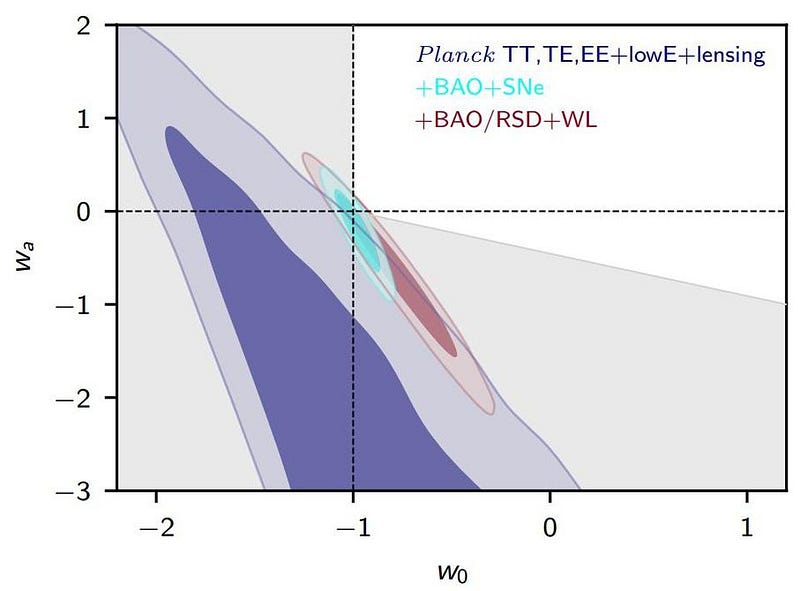
There’s also the question of whether dark energy is truly a cosmological constant or not, which is extremely sensitive to both the CMB and the data from the ultra-distant Universe, such as Type Ia supernovae. If dark energy is a perfect cosmological constant, its equation of state, given by the parameter w, should equal -1 exactly.
The measured value?
We find that w = -1.03, with an uncertainty of 0.03. There is no evidence for anything else, meaning that both the Big Crunch and the Big Rip scenarios are not favored by the data.
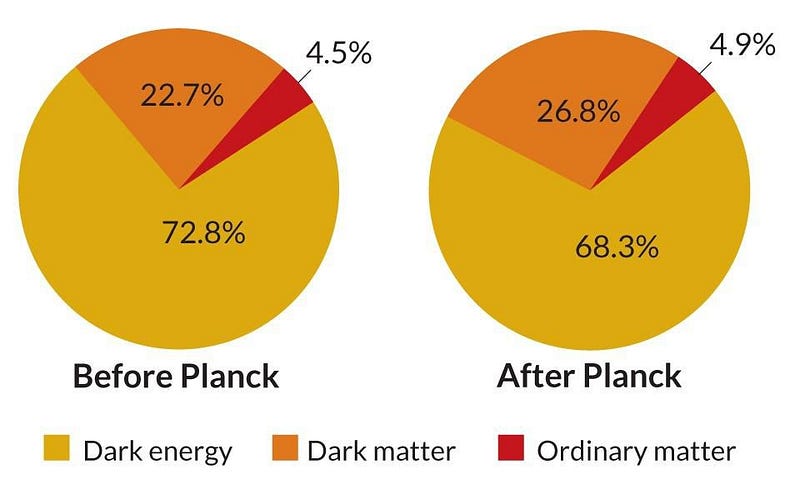
Other quantities have changed mildly. The Universe is a little older (13.8 instead of 13.7 billion years) than we previously thought; the distance to the edge of the observable Universe is a little smaller (46.1 instead of 46.5 billion light years) than WMAP had indicated; the constraints on the strength of the gravitational wave signal generated by inflation are a little bit better than they were previously. A parameter known as the tensor-scalar ratio, r, had been constrained to be less than 0.3 before Planck. Now, with Planck data, large-scale structure data, and other experiments (like BICEP2 and the Keck Array) weighing in, we can confidently state that r < 0.07. This rules out a few models of inflation that could have been considered viable previously.
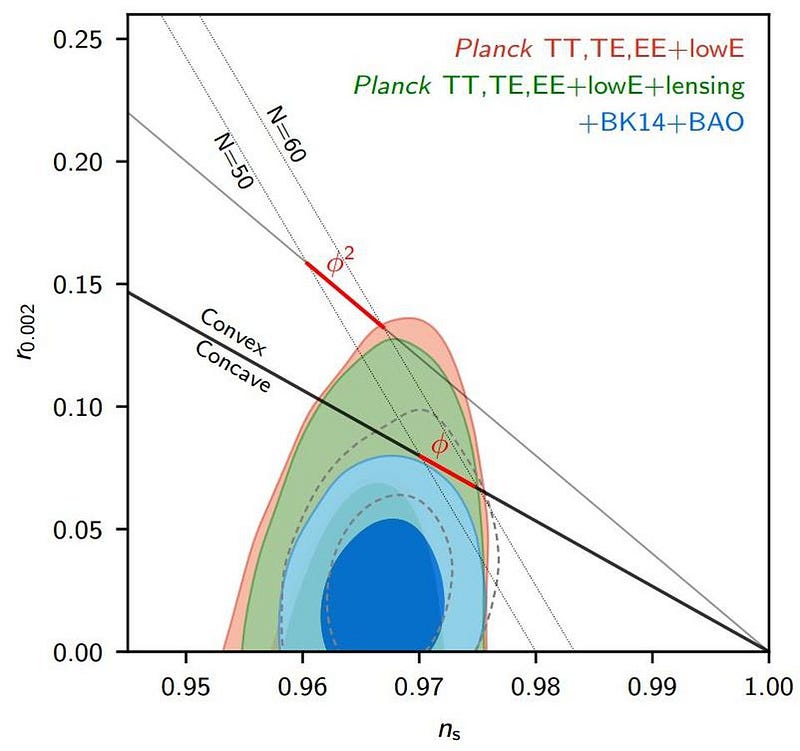
So, with all the data in, what can we say yes-and-no to when it comes to the Universe and what it’s made of?
- Yes to inflation, no to gravitational waves from it.
- Yes to three very light, standard-model neutrinos, no to any extras.
- Yes to a slightly slower-expanding, older Universe, no to any evidence for spatial curvature.
- Yes to a little bit more dark matter and normal matter, yes also to a little less dark energy.
- No to changing dark energy; no to the Big Rip or the Big Crunch.
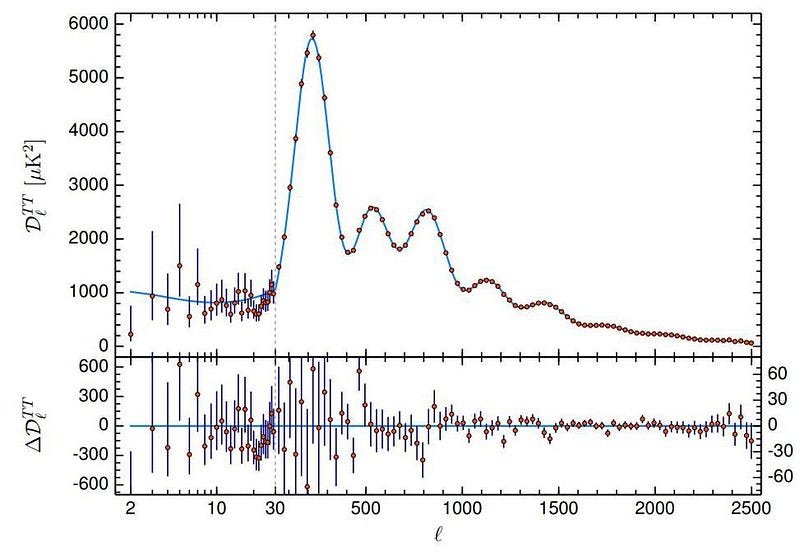
Most importantly, a spectacular agreement to a never-before-achieved precision now exists between the CMB we observe and the theoretical predictions of a Universe with 5% normal matter, 27% dark matter, and 68% dark energy. There might be wiggle room of 1–2% in some of those numbers, but a Universe without dark matter and dark energy, both, in great abundance, is a no-go in the face of these observations. They’re real, they’re necessary, and their predictions match the full suite of data perfectly.
Inflation, neutrino physics, and the Big Bang have additional pieces confirming them, while alternatives and specific variants are better constrained. Most definitively, the Planck collaboration states, “We find no compelling evidence for extensions to the base-ΛCDM model.” At last, we can state, with extraordinary confidence, what the Universe is made of.
Ethan Siegel is the author of Beyond the Galaxy and Treknology. You can pre-order his third book, currently in development: the Encyclopaedia Cosmologica.