LIGO’s Biggest Mass Merger Ever Foretells A Black Hole Revolution
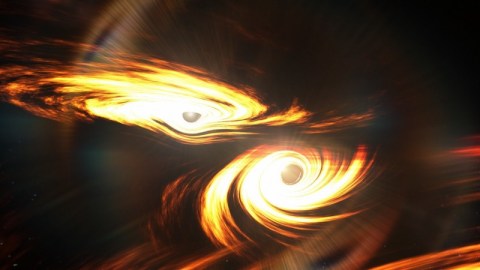
When two black holes that shouldn’t exist are seen merging, physics has some explaining to do.
After years of searching for gravitational waves, it finally happened: LIGO bagged the biggest one ever. Approximately 10 billion years ago, two massive black holes — weighing in at 85 and 66 times the mass of our Sun — merged together, converting approximately 8 solar masses into pure energy in the form of gravitational radiation. After journeying through the expanding Universe, those signals arrived at the National Science Foundation’s LIGO and the European Gravitational Observatory’s Virgo detectors, where they were detectable over a timespan of just ~13 milliseconds. It was the most massive black hole merger ever detected.
It’s remarkable for a number of reasons, as it sets a slew of records, including:
- the most distant black hole-black hole merger (at 17 billion light-years away, accounting for the Universe’s expansion),
- the most massive progenitor black holes (at 85 and 66 solar masses),
- the most massive final black hole (at 142 solar masses),
- the greatest amount of mass turned into energy in a single event (8 solar masses),
- and the shortest-duration definitive signal ever seen (at ~12.7 milliseconds).
But the biggest surprise of all is that we didn’t expect these black holes to exist at all. Here’s the enormous puzzle presented by this new discovery, and the leading ideas on what the solution might be.
The way gravitational wave detectors like LIGO actually “see” merging black holes is that these mergers create ripples in spacetime, where space alternately compresses and expands in two perpendicular directions, in phase, as the gravitational waves pass through them at the speed of light. By creating a detector where light travels repeatedly down-and-back along two long-baseline arms in perpendicular directions, those small and periodic distance changes can be seen, down to even a tiny fraction of a wavelength of the light used. Mirror displacements as small as ~10^-19 meters can be detected.
But we can’t detect every source of gravitational waves in the Universe: only the ones that have both a sufficiently large amplitude (creating a large enough change in the relative positions of the mirrors) and that fall into a frequency range that the detectors are sensitive to (based on the physical size of the detector’s arms). Ground-based detectors like LIGO and Virgo are sensitive to mergers of collapsed objects — black holes and neutron stars — ranging from a few solar masses up to perhaps a few hundred solar masses.
This newest event, now officially known as GW190521, is the heaviest black hole-black hole merger ever seen. It’s so massive — and therefore, its event horizon is so large — that only the last couple of orbits before the merger could be seen by our terrestrial detectors. The “ringdown” phase, where the post-merger black hole settles down, could actually be detected as well, which provides a phenomenal amount of information to gravitational wave scientists about the properties of this merger. It really is this massive, this distant, and inconsistent with being anything other than two black holes merging together from nearly perfectly circularized orbits.
The post-merger black hole, at 142 solar masses, is also the very first “intermediate mass black hole” ever detected. We’ve detected stellar mass black holes before, which we loosely categorize as under 100 solar masses, which are assumed to form from massive stars that go supernova, experience a catastrophic instability, or otherwise collapse entirely. We’ve also detected supermassive black holes: of 100,000 solar masses or more, which live at the centers of massive galaxies. But for the in-between black holes, this is the first.
Based on the black hole-black hole mergers already seen by LIGO and Virgo, we’d already learned an important lesson: 99% of black holes in binary, merging systems are below 43 solar masses. This is, at least so far, the first and only black hole-black hole merger we know about where both members are above that ~43 solar mass threshold. It’s an important milestone for a vital reason: there must be some way to build up these supermassive black holes from smaller black holes, and that requires a population of these intermediate mass black holes. At last, we’ve discovered the very first one.
We know how the first one we’ve ever seen arose: from the merger of two lower-mass black holes. We don’t know if mergers, accretions, or some other mechanism (such as the direct collapse of material) is responsible for the majority of these intermediate mass black holes that must exist in the Universe, but at least we know how the first one came about. What we don’t know, however, is how we physically created at least one of the black holes — the 85 solar mass one — that led to its formation.
In theory, the lower-mass black holes are called “stellar mass” black holes because they arise as the remnants of stars, which live, die, and leave a black hole remnant behind. For all of the previous black holes seen by gravitational wave detectors, this explanation worked just fine, as the theoretical predictions for how massive stars died lined up with our observations of the black holes that existed.
But an 85 solar mass black hole? That, according to our best current understanding of stellar evolution, shouldn’t be possible.
Here’s why: if a star is massive enough to go supernova, it will form either a neutron star or a black hole, depending on its original mass. In general, the more massive a star is, the more massive the remnant it leads to. But this only works up to a point. Above a certain mass, the temperature inside the star gets so hot — above about 3 billion K — that the most energetic photons, which provide the radiation pressure that hold the star up against gravitational collapse, can spontaneously convert into matter-antimatter (electron-positron) pairs. This is a disaster for the star.
When this radiation spontaneously becomes matter and antimatter, it causes the radiation pressure inside the star to plummet, which lets gravitational collapse gain the upper hand. As a result of this collapse, the interior of the star gets even hotter: the same way that rapidly compressing a gas can cause it to heat up. This converts even more photons into electron-positron pairs, and this continues until a runaway fusion reaction is triggered in the star’s core, causing it to go supernova. Astrophysicists call this a “pair-instability supernova,” and it leads to the destruction of the entire star, with no remnant left behind.
Unfortunately, that should basically forbid the existence of stellar mass black holes in a certain mass range, and that range should definitely include an 85 solar mass black hole. The fact that LIGO and Virgo saw this merger with the properties they did indicates very strongly that — despite our theoretical expectations — black holes in this “forbidden” mass range really do exist. The big new question that arises as a result of this finding is simply: how?
1.) Our understanding of high-mass stellar interiors is incorrect. Maybe the pair-instability mechanism doesn’t work as we suspect. Maybe there’s some new physics we haven’t considered. Maybe neutrino production carries energy away and leads to black hole formation. Or maybe metallicity (the fraction of heavy elements in a star) can change this equation. It seems unlikely because the science is so theoretically well-understood, but we always have to consider that we might have something wrong.
2.) These black holes weren’t formed from stars, but are primordial: left over from the Big Bang itself. This is one of those extraordinarily unlikely scenarios with no evidence in favor of it, but not quite enough evidence to rule it out entirely. It’s possible that, in the early Universe, there were regions of space with more matter than average, and they collapsed directly to form black holes. That would require a region with ~68% or more “extra” matter in it compared to the average; the largest overdensities we know of are of ~0.01% in magnitude. It’s not likely, but we can’t fully rule it out.
3.) These black holes weren’t formed from the death of a single star. Now we’re starting to get into the realm of actual possibility here. We know that 50% of all stars form as a part of multi-star systems, and that a substantial fraction of stars (more than 10%) live in systems with 3, 4, 5, 6, or even 7 stars in them. (More are possible, but we haven’t found them yet.) If two or more stellar mass black holes merged together to create these progenitor black holes, which then merged in this event, there’s no problem at all. The biggest challenge to this scenario may be understanding why, when the earlier merger(s) occurred, the other members weren’t ejected in the process!
4.) These black holes grew after accreting mass from (or swallowing) a companion. They say that “might makes right” in warfare, and in astrophysics, a similar analogy is true. The highest-mass and highest-density clumps draw in the matter around them, and if these black holes formed with companions, some or even all of that matter could have been swallowed by the black hole after they formed. It’s a way for these black holes to grow to these higher masses without needing to form, immediately, with this supposedly forbidden mass values.
5.) These black holes formed within the accretion disk around an active supermassive black hole. This is a wild scenario, but it may actually turn out to be correct. One of the places we know we’re likely to find black holes merging together is near the centers of galaxies, as matter often falls in towards the central black hole. These dense regions frequently have lots of new stars forming in them; we see this even in our own galaxy. When a large amount of matter nears the central black hole, it can become active, creating an accretion disk, a region with a lot of drag, and “flares” when black holes merge together, either with one another or with the central black hole.
In an environment such as this, a black hole can easily accrete lots of mass, growing substantially in this environment. The 85 and 66 solar mass black holes may have been significantly smaller when they formed, having grown within the accretion disk. There is some exciting potential evidence for this, as an electromagnetic flare was seen coincident in time (and possibly in space) with this gravitational wave merger. Even if the observed flare is unrelated, this scenario still remains plausibly viable.
In many ways, this is the best kind of science: an observation that surprises us, and compels us to rethink our theoretical assumptions in the process. We’ve just witnessed the heaviest black hole-black hole merger ever directly seen, and that led to the first definitive detection of an intermediate mass black hole of all. This event set a number of records, and ranks as the single most energetic event ever witnessed since the Big Bang: unleashing more than 100 times the energy of all the stars in the Universe over a brief period of ~13 milliseconds.
It also raises a number of spectacular questions. How did the black holes that gave rise to this intermediate mass one form? Do most intermediate mass black holes form this way, or from a different mechanism? Are these black holes currently embedded in the accretion disk of an active galaxy? Did they “flare” when they merged, and did we see it? Now that we’ve seen our first one, we can be certain these objects are out there. As additional observations take place and new data comes in, we can look forward to answering questions that, just a few short days ago, we didn’t even know we should be asking.
Ethan Siegel is the author of Beyond the Galaxy and Treknology. You can pre-order his third book, currently in development: the Encyclopaedia Cosmologica.