A new origin story for supernovae after 94 years
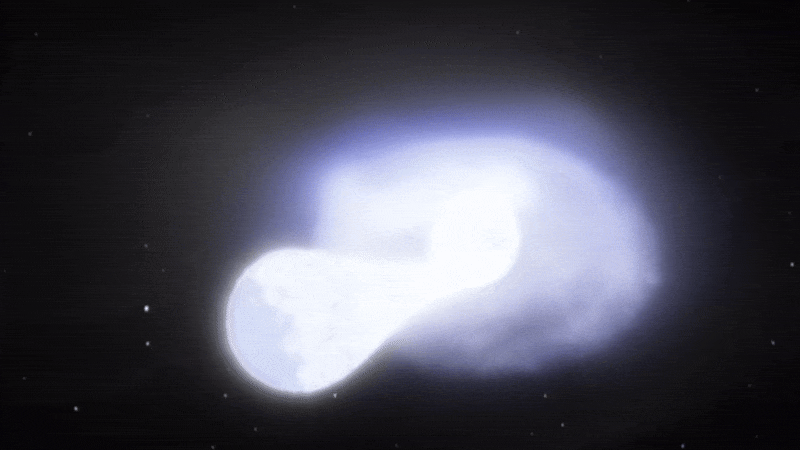
- Since the 1920s and 1930s, scientists have recognized that there’s a maximum mass that certain types of matter can have before they inevitably collapse, implode, or are otherwise destroyed.
- The observations of type Ia supernovae indicate that they come from white dwarfs that explode, leading most to connect the 94-year-old Chandrasekhar mass limit with these stellar cataclysms.
- However, research in recent years suggests that most type Ia supernovae instead arise from a very different scenario: double detonation. Here’s how the science is both improving and changing.
Whenever a star is being formed, there will be two opportunities throughout its life to go supernova. The first comes early on: if the star is massive enough, when it exhausts first the hydrogen and next the helium within its core, it can continue onward and fuse carbon, neon, oxygen, and then silicon in succession, until the core at last implodes, resulting in a core-collapse (type II) supernova. If the star isn’t massive enough to succumb to that fate, it will blow off its outer layers after helium-burning and form a planetary nebula, while the core contracts to form a white dwarf. If that white dwarf then experiences the right conditions, its interior will detonate, producing a different (type Ia) class of supernova: the most distant “standard candle” known in astronomy.
Since the 1920s and 1930s, it’s been known that — above a certain mass threshold — white dwarfs would become unstable against gravitational collapse. The presence of electrons, and the fact that no two electrons in the same system can ever occupy the same quantum state, is what prevents white dwarfs from imploding. However, above a mass threshold known as the Chandrasekhar mass limit, that electron degeneracy pressure is suddenly too weak, leading to a cataclysm for the white dwarf remnant.
For roughly the past 94 years, the leading story was that type Ia supernovae are caused by white dwarfs that gain enough matter to surpass this limit, triggering their explosion. Over the past two decades, however, research has shown that this story is largely insufficient. Instead, the double detonation scenario, developed in detail in recent years, provides a new origin story for these events. It’s now time for the science to evolve as well.

We have to recognize multiple facts about white dwarf stars before we dive into the detonation events themselves. To begin, white dwarfs arise from the cores of massive stars that do burn through all of the hydrogen present within them, but are (typically) unable to burn elements heavier than helium, even after the core gravitationally contracts and heats up. (Although, in some exotic cases, stars can initiate carbon, neon, or even oxygen-burning in their cores, and still become a white dwarf if a binary companion steals enough mass away.) In most scenarios, the end stage that produces a white dwarf occurs when the outer layers of the evolved star, containing mostly hydrogen and helium, are gently blown off, while the remnant core, consisting mostly of carbon-and-oxygen, contracts due to gravity.
While those blown-off layers are returned to the interstellar medium, the contracting core reaches a boundary: the fact that the quantum particles that compose it — specifically the electrons — get compressed into a smaller and smaller volume, such that their wavefunctions begin to overlap. Because electrons are fermions, a species of particle where no two of them can occupy the same quantum state, there’s going to be a special (quantum-in-nature) type of pressure that pushes back against gravitational collapse: quantum degeneracy pressure. So long as this object, a white dwarf, remains below the Chandrasekhar mass limit, this degeneracy pressure should be sufficient to prevent further collapse.

Realistically, most of the white dwarfs that have been observed to form in the Universe are not close to the Chandrasekhar limit, but are substantially below it. White dwarfs typically range from about 0.5 solar masses up to about 1.1 solar masses, with only a few known white dwarfs of ~1.2 or ~1.3 solar masses known. However, white dwarfs aren’t just the end-state for stars like our Sun, but are the end-state for most stars born with between 0.4 and 8 solar masses worth of material. While about 50% of such stars are found in singlet systems, like our own, the other 50% are members of multi-star systems, with binaries being the most common configuration.
Binary star systems, importantly, often form with similar masses, meaning that if one (more massive) star in the system is going to die in a planetary nebula/white dwarf combination, then the other star shouldn’t be far behind, and should follow a similar evolutionary track. Because of how stellar evolution works, we know that stars:
- first begin fusing hydrogen in their cores,
- then, when their hydrogen is exhausted, expand into red giants and begin fusing helium,
- and afterward, when their helium is exhausted, they blow off their outer layers and become white dwarfs.
Therefore, the conventional story goes, a dense white dwarf remnant with an evolved, giant companion will begin siphoning mass off of its less dense companion until a critical threshold is reached: one where the mass of the accreting white dwarf crosses over the Chandrasekhar limit. And that, therefore, is how type Ia supernovae occur.
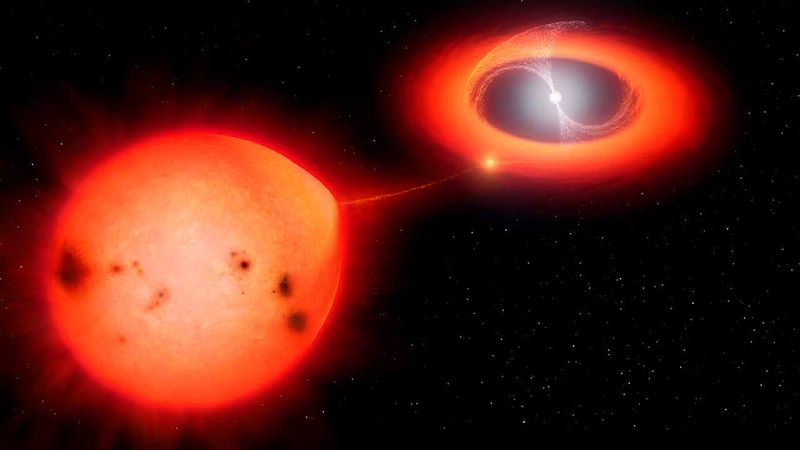
This is an example of a straightforward, simple story that makes sense, obeys all of the known laws of physics, and yet is completely wrong. This scenario absolutely does not explain the type Ia supernovae we observe, because if it did, then all type Ia supernovae would have the same mass upon detonation: a mass that just barely crossed over the threshold of a mass limit. That would lead to the same physics underlying each and every such type Ia supernova, which should lead to what astronomers call the same light-curve: where the brightness rises, peaks, and then falls off over time with the same intrinsic amplitude and at the same intrinsic rate all across the Universe.
But that idea has never been consistent with what we’ve observed.
Instead, type Ia supernovae follow a variety of light-curves, with more luminous type Ia supernovae taking longer to reach peak brightness and then taking longer to decline from that peak brightness, while less luminous varieties reach their peak brightness earlier and fade away more swiftly. In order to explain this, something needs to be different about these supernovae from one another: not just between the environments where they occur, but intrinsically as well. In other words, it doesn’t make sense, astrophysically, if these type Ia supernovae all occur with the same mass on the same type of object crossing over the same threshold. Something about them must be different from one another.
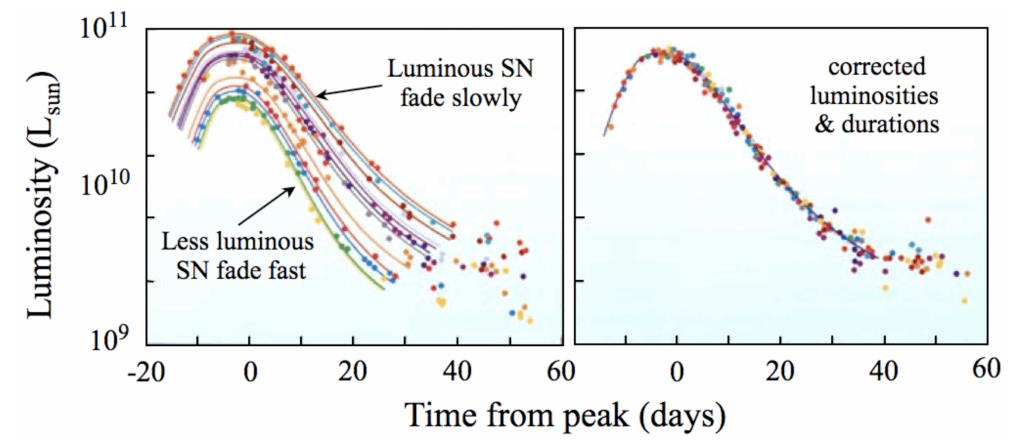
One alternative scenario has been considered for a long time: that instead of detonating when a large amount of mass is accreted onto a white dwarf, a white dwarf detonation could be triggered by colliding and/or merging with another white dwarf. After all, we only need to move one stage further ahead in the stellar evolution of a binary system to get there; if a white dwarf can persist through its companion star’s red giant phase, then that red giant will blow off its outer layers and contract down to form a white dwarf itself. When these two white dwarfs then merge with one another, their combined mass would exceed the Chandrasekhar mass limit, and a detonation would be inevitable.
As Chandrasekhar himself noted way back in 1939, it’s the stability or instability of the electrons in the white dwarf’s core that will determine whether it remains intact or not.
“If the degenerate core attains sufficiently high densities, the protons and electrons will combine to form neutrons. This would cause a sudden diminution of pressure resulting in the collapse of the star to a neutron core.”
However, Chandrasekhar got this one wrong: before reaching those densities, nuclear fusion reactions occur between carbon atoms inside the core: a fusion reaction that occurs before electron capture ever begins. As a result, after around ~1000 years of carbon fusion, the original white dwarf is destroyed by a runaway reaction, leaving no remnant behind at all.
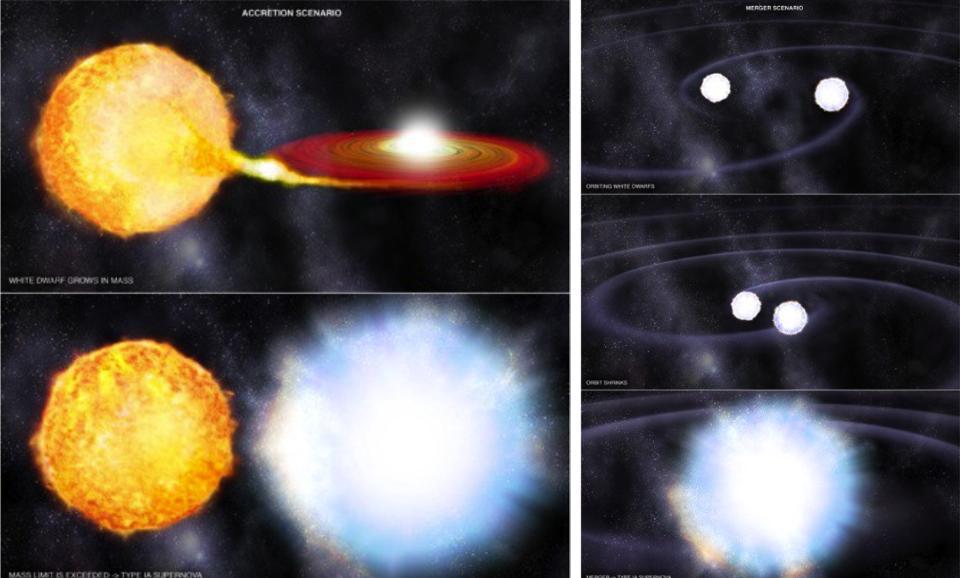
Unfortunately, even adding in this extra element is too simple of a fix to explain what we observe, too! There are other complications that the simple models gloss over, and which we have to include. For one, white dwarfs aren’t just solid balls of degenerate matter, composed of heavy elements like carbon (and up), but rather have light-element atmospheres atop them, where a shell of helium and even (potentially) hydrogen are present as well. When enough matter accumulates on the surfaces of these white dwarfs (which does frequently occur through accretion from a binary companion), a plain old regular nova can occur: where nuclear fusion ignites in this outer shell, causing a temporary brightening due to these reactions before fading away, and taking anywhere from years to millennia to recharge.
The difference between an accretion event that produces a nova and an accretion event that produces a supernova was long thought to only depend on how massive the progenitor white dwarf was: near the Chandrasekhar mass limit, you can get a supernova, but far from it, you’ll just get a regular nova. However, there’s another possibility besides a regular (subsonic) nova for these white dwarfs — irrespective of whether they accrete matter or merge/collide with another astrophysical body — and that’s where a nuclear fusion reaction is indeed triggered on the white dwarf’s surface resulting in a detonation, where a non-burning shock wave then propagates successfully into the core, which can then trigger another detonation (set of nuclear reactions) in the core: destroying the white dwarf in a runaway reaction that is indeed a type Ia supernova.
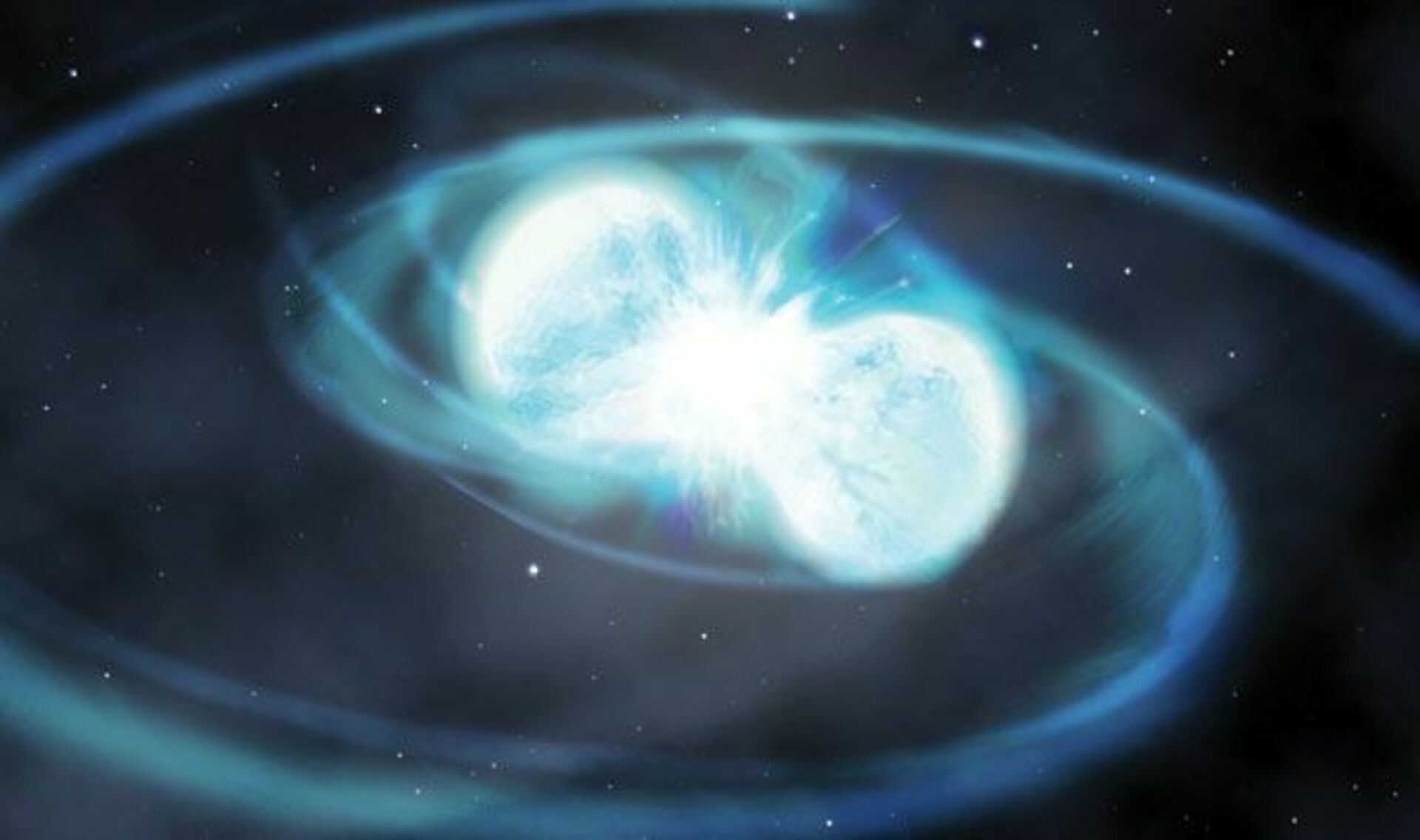
That is, at its heart, what the double detonation scenario is all about. It isn’t that there’s a gradual or even an abrupt change in the overall mass of the star that causes gravitational pressure to increase, triggering a detonation in the core that causes a type Ia supernova. The ways in which that idea fails are plentiful:
- there’s too little massive material in these supernova remnants to add up to something that reached/exceeded the Chandrasekhar limit,
- there’s too much variation in supernova brightnesses and light curves to explain a detonation with universally identical masses and processes,
- and the white dwarfs that we actually find in nature are almost always well below the Chandrasekhar mass limit, making it difficult to explain how such large numbers of type Ia supernovae are seen when there are so few white dwarfs that even approach 1.4 solar masses.
Instead, the double detonation scenario takes a white dwarf that’s well below the Chandrasekhar mass limit — of a stellar remnant that’s only around 1.0 solar masses total — and considers a detonation on a light helium-dominated shell, arising either from a prior accretion event, a merger with another white dwarf, or simply having been born with enough helium to initiate this. With a carbon-oxygen core of around ~0.8-1.1 solar masses, a typical white dwarf’s core will receive a large enough shock from such a surface detonation to trigger its internal detonation as well: destroying the star entirely.

A double detonation scenario for white dwarfs in type Ia supernovae fits the data much better. The matter found in all of the ejecta from a type Ia supernova better matches up with lower white dwarf masses rather than Chandrasekhar-like ones, and these sub-Chandrasekhar mass white dwarfs are the ones found in great abundance throughout the galaxy and Universe, rather than the ultra-rare ones that approach the Chandrasekhar mass limit. Additionally, a variety of masses, particularly of carbon-oxygen core masses, for the progenitor white dwarfs can better explain the variety of supernova light-curves that have already been observed. The puzzles that cannot be answered by white dwarfs that all cross over the Chandrasekhar limit can all be solved by a double detonation scenario.
But there’s another observation that could be serendipitously explained by double detonation scenarios: a class of hypervelocity supernova survivors. Back in 2018, using data from the Gaia satellite that surveys stars and stellar remnants within the Milky Way, scientists (led by Ken Shen, who discussed the double detonation scenario previously on the Starts With A Bang podcast) identified three white dwarf stars that appeared to have enormous velocities: over 1000 km/s and in some cases approaching 3000 km/s, or 1% the speed of light. These speeds are much, much greater than the escape velocity of the Milky Way, so something must have “kicked” them relatively recently to give them these fast motions. Once enough time has passed (just another few tens of millions of years), these white dwarfs will be in intergalactic space.
Where did they come from?
Remarkably, as argued in a new paper that was recently accepted for publication in The Astrophysical Journal, the most likely answer is from the merger of two white dwarfs. When white dwarfs are about to merge, there’s an excellent chance that there will be a surface detonation on one of them, and there’s also an excellent chance that surface detonation will trigger a core detonation in the aftermath: the double detonation scenario.
However, there’s now a second white dwarf to consider as well. There’s a chance that white dwarf, too, will undergo a double detonation, as ejecta from the type Ia supernova will impact the white dwarf, triggering a fusion reaction that could propagate to the core, leading to a double-double detonation. Some secondary white dwarfs, however, might not double detonate, but instead:
- undergo only a set of surface reactions that do not result in shock waves propagating into the core,
- get ablated and mass-stripped from interacting with the supernova and its ejecta,
- while being kicked to great speeds by slingshot effect of the primary white dwarf’s destruction.
This could be responsible for the hypervelocity white dwarfs we’ve seen, including one such example where the white dwarf appears to be moving directly away from an ancient supernova remnant.
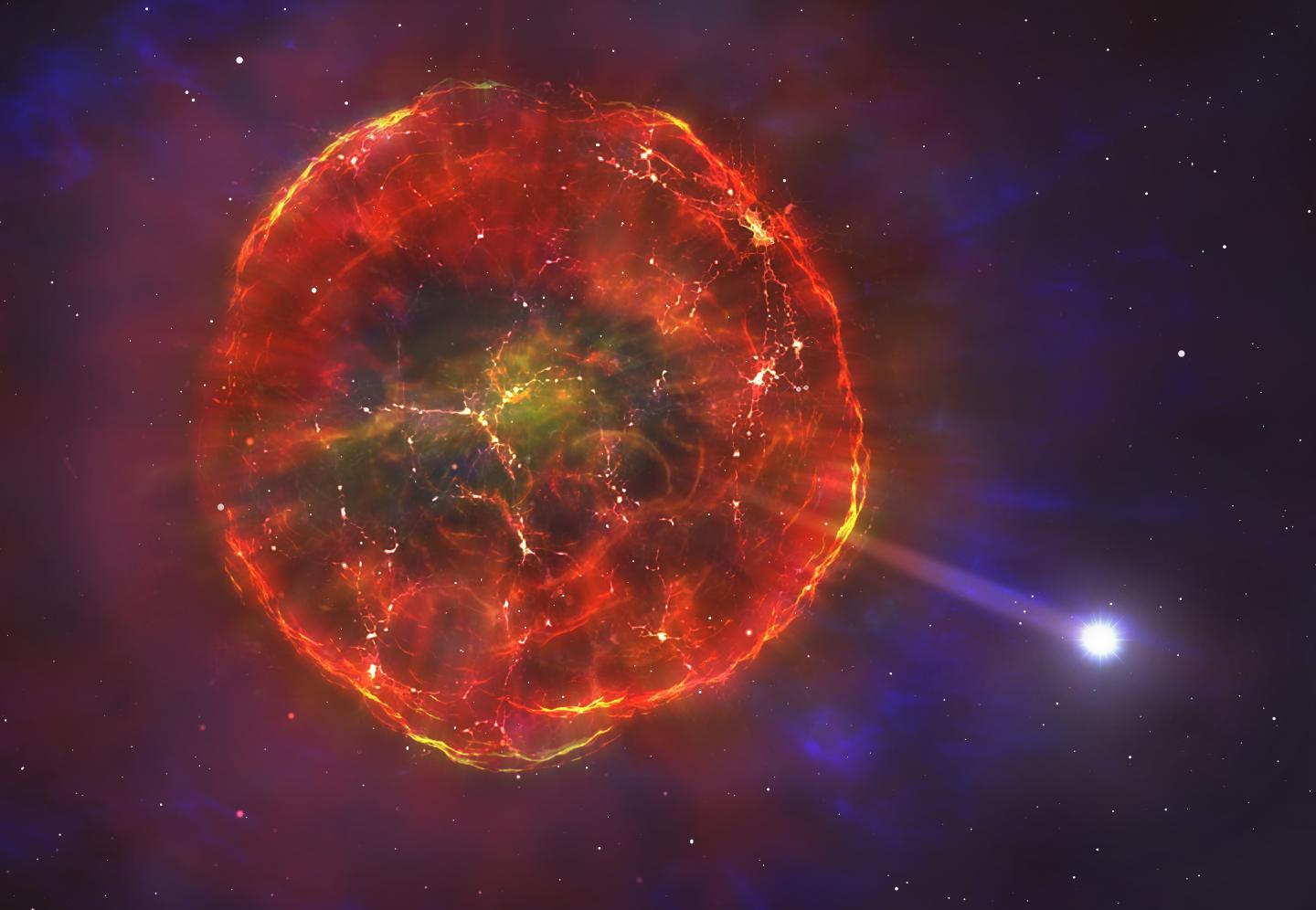
However, not all hypervelocity white dwarfs are necessarily “smoking guns” of a double detonation event. For example, there’s a very unusual white dwarf known as SDSS J1240+6710. This white dwarf has a very unusual atmosphere: no hydrogen or helium, as well as no iron, nickel, chromium, or manganese: common features of all thermonuclear supernovae. Instead, elements like carbon, oxygen, neon, magnesium, silicon, sodium, and aluminum are all abundant, indicating that a surface thermonuclear reaction took place. Additionally, the white dwarf is exceptionally low in mass: just 40% the mass of the Sun, all while speeding through the galaxy after being kicked at an estimated ~500 km/s.
Is it a white dwarf that survived being in very close proximity, initially, to a white dwarf that did indeed undergo double detonation? It turns out the answer is “probably not,” as this may be another example of the rare class of type Iax supernovae: like SN 1181 is strongly suspected to be the remnant of. We must be careful not to assume that every hypervelocity white dwarf arose from the double detonation of a companion.
If the double detonation scenario holds up — and the evidence keeps pouring in in support of it and against the traditional “Chandrasekhar limit” scenario — it could mean that double detonations might be not only the dominant mechanism for creating type Ia supernovae within the Universe, but such a mechanism might potentially explain all the “normal” type Ia supernovae ever observed. For most of the 20th century and even well into the 21st, astrophysicists have been teaching the Chandrasekhar mass limit as the key threshold when it comes to white dwarf stability. With the advent and rise of the double detonation scenario, it may at last be time to retire this outdated story for how type Ia supernovae actually ignite!