Ask Ethan: Are singularities physically real?
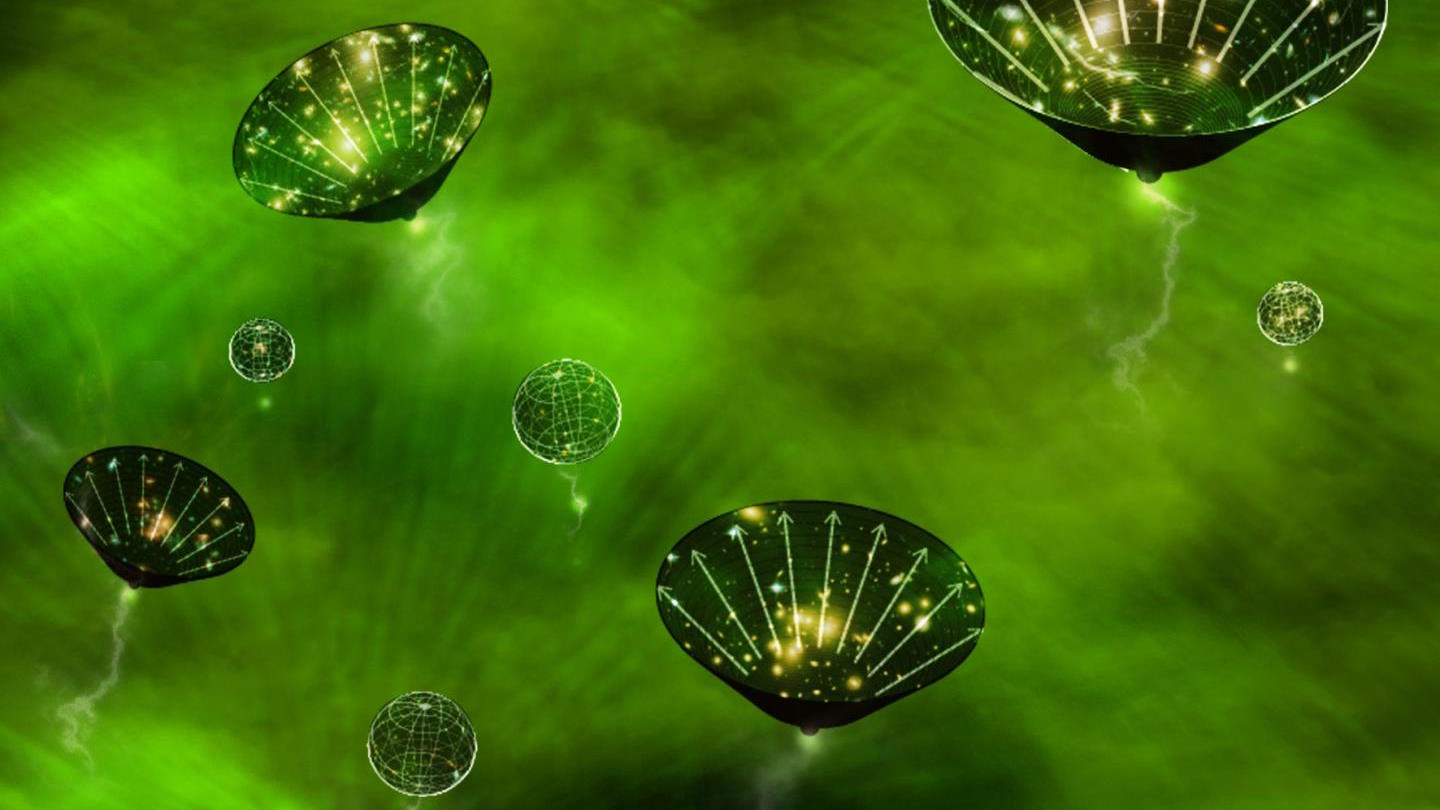
- Wherever you have too much mass-or-energy together in one location in space, you inevitably arrive at what’s known as a singularity: a place where the laws of physics break down.
- This occurs because Einstein’s general relativity and the small-scale quantum universe don’t play nice together, and predictions under those physical conditions no longer make sense.
- However, are singularities physically real in some sense, or are they just an indication that something else, such as a quantum theory of gravity, is required? It’s time to unpack what we know.
One of the most important advances in all of physics was the development of Einstein’s general relativity: our greatest and most predictively powerful theory of gravity. Replacing the idea of a “gravitational force” that acts on objects that never physically touch one another with the notion that all objects exist within the fabric of spacetime, and that the curvature of spacetime determines how those objects will move, is a concept that many — even professionals — still struggle to wrap their heads around. However, it comes along with consequences: certain configurations of matter-and-energy within spacetime inevitably lead to a condition that marks an effective “end” or “beginning” to spacetime itself, more commonly known as a singularity.
But are these singularities necessarily physically real, representing something profound that’s occurring within the Universe? Or might there be some way to avoid them, perhaps signaling a very different scenario than space and time themselves ceasing to exist? (At least, as we understand them.) That’s what Patreon supporter Cameron Sowards wants to know, as he writes in to ask:
“Why do we believe that the pre big bang state was not a singularity when it is a much higher concentration of energy than a black hole could possibly have… since the pre big bang universe was not a singularity, could the same mechanisms that prevented it from being a singularity apply to the interior of black holes?”
There’s a tremendous amount to unpack here, so let’s try and do this question justice!
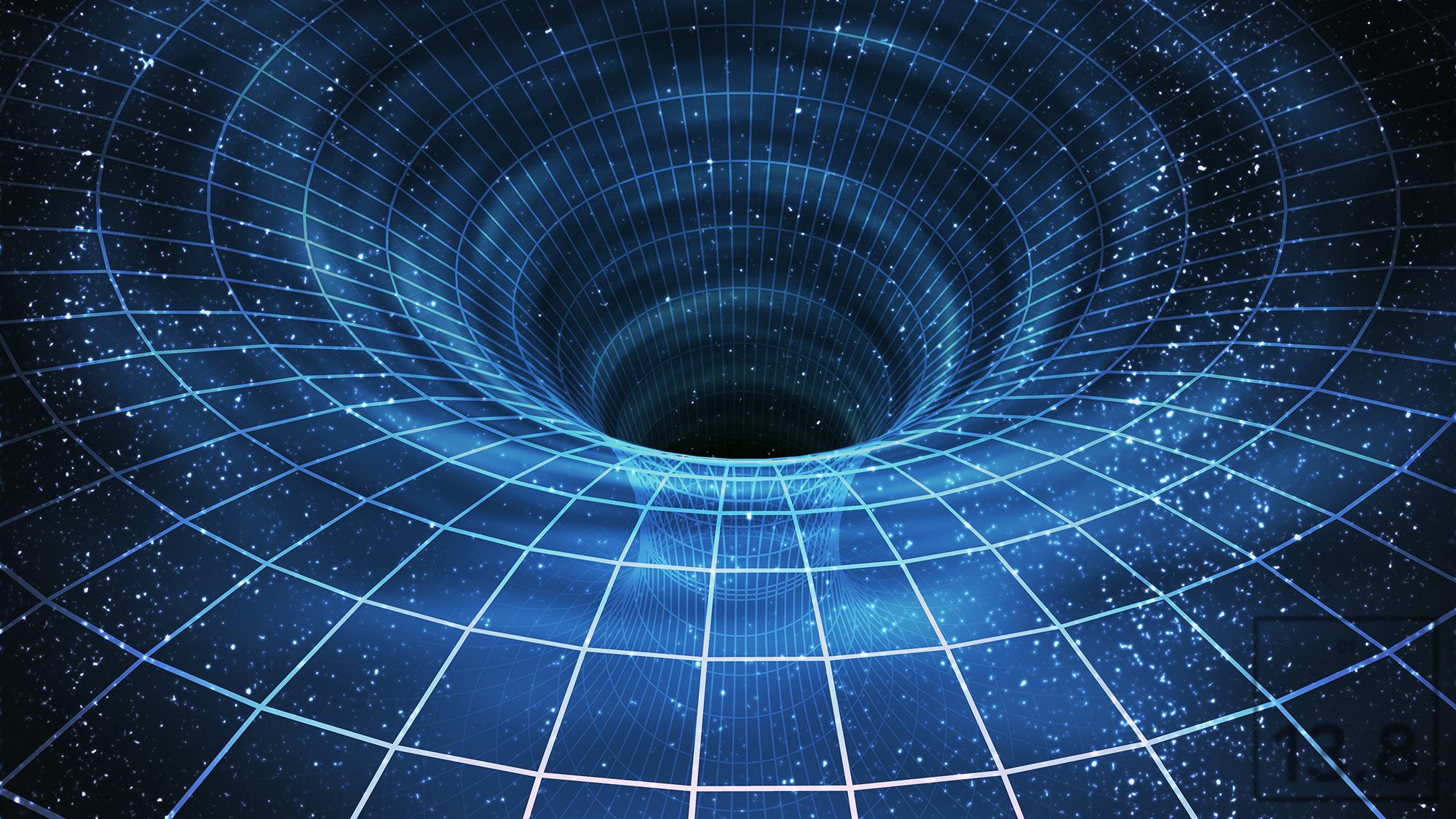
The Big Bang and the question of a “first” singularity
If you start with just two basic observations — that the Universe is full of matter and energy, and also, is expanding today — you might think there’s no way out of an initial singularity. Indeed, this was first put together nearly a hundred years ago, all the way back in the 1920s. As soon as you recognize that your Universe, on the largest of cosmic scales, is roughly the same in all locations and in all directions (what astrophysicists call “homogeneous” for the first and “isotropic” for the second), then there’s a particular exact solution (and metric for spacetime) that applies within the context of general relativity: the FLRW (Friedmann–Lemaître–Robertson–Walker) metric.
This metric, which describes the spacetime of the Universe as well as its relationship to the matter and energy within it, mandates that the Universe cannot be static, but must either expand or contract. Given that observations of the recession speed (or redshift) of distant galaxies is directly proportional to their measured distance from us, this indicates that the Universe is expanding today.
If it’s expanding today, and full of matter and radiation, then that implies that in the past, the Universe was smaller but contained the same amount of “stuff” within it. Therefore, it was denser and hotter as well. The farther we extrapolate back in time, the smaller the Universe gets. And if we go all the way back to the moment where it reaches “0” for its size, we arrive at a singularity.

This picture held sway for most of the 20th century, having been bolstered by what are known as the four observational cornerstones of the Big Bang theory.
- The observation that the Universe is expanding, as shown most clearly by the redshift-distance relation discovered by Lemaître (in 1927), and then later by Robertson (in 1928), and then later again by Hubble (in 1929-1931).
- The formation and growth of cosmic structure in the Universe: from an early, roughly uniform state to a clumpier, more clustered state consisting of stars, galaxies, galaxy groups and clusters, and a filamentary cosmic web at later times.
- The existence and blackbody spectrum of a cosmic microwave background: a background of leftover radiation dating back to the hot Big Bang itself, from an epoch when the early Universe was too hot for neutral atoms to stably form; once the atoms do form, the radiation gets released, and we can observe it today.
- And finally, the abundance of the lightest elements and isotopes of all: hydrogen, deuterium, helium-3, helium-4, and a tiny amount of lithium-7, all forged in the crucible of the hot Big Bang, before any stars could form.
With these four pillars supporting the hot Big Bang, there was no doubt that this theory — in contrast to all other competing models — accurately describes our cosmic origins.
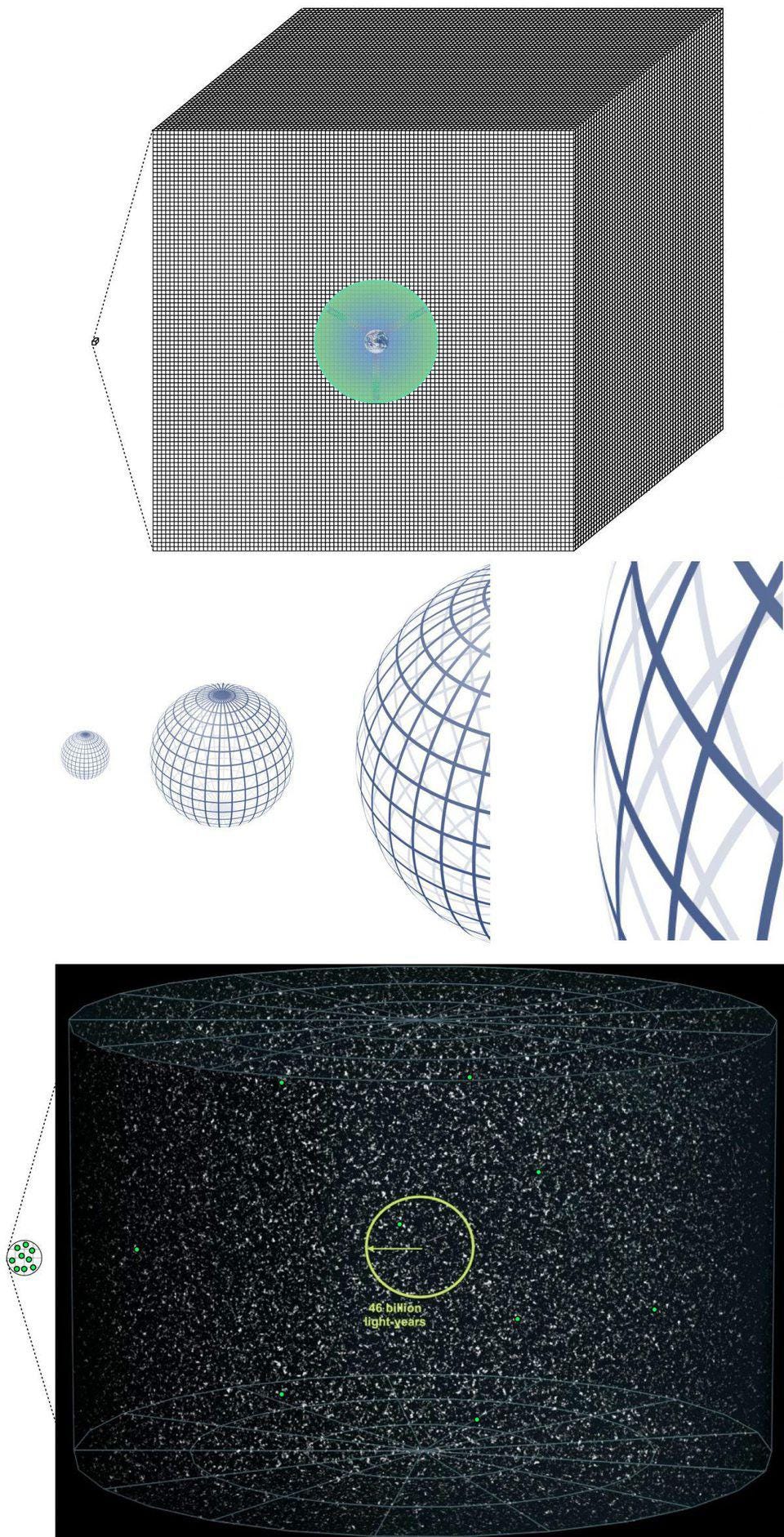
But just because this story describes our past doesn’t necessarily mean it’s “chapter 1” of the story of our Universe. There are a great many unexplained puzzles that come along with the hot Big Bang, including:
- Why, if the Universe reached incredibly high temperatures, are there no high-energy relics from those epochs still around in our Universe today? (Historically known as “the monopole problem.”)
- Why, because of how cosmic expansion works, was the Universe born with its expansion rate and its total energy density perfectly balanced, so that even billions of years later, it’s still perfectly spatially flat? (Historically known as “the flatness problem.”)
- And why, when we look at different regions of the sky that have not had time to exchange information or signals with one another, even at the speed of light, do they appear to be in perfect thermal equilibrium? (Historically known as “the horizon problem.”)
In the standard hot Big Bang, there are no explanations for this. You have to simply assert that “these are the initial conditions of the Universe” with no explanation, or as Lady Gaga might say, the Universe was simply “born this way.”
However, there’s a wonderful scientific mechanism that can set up these conditions if we hypothesize an early phase to the Universe that preceded the hot Big Bang: cosmological inflation. This theory, first proposed in 1980, not only provides explanatory power for all three of these observations, it also made an incredible new set of predictions that differ from that of a hot Big Bang without inflation, including some really weird ones, that have since been observationally confirmed.
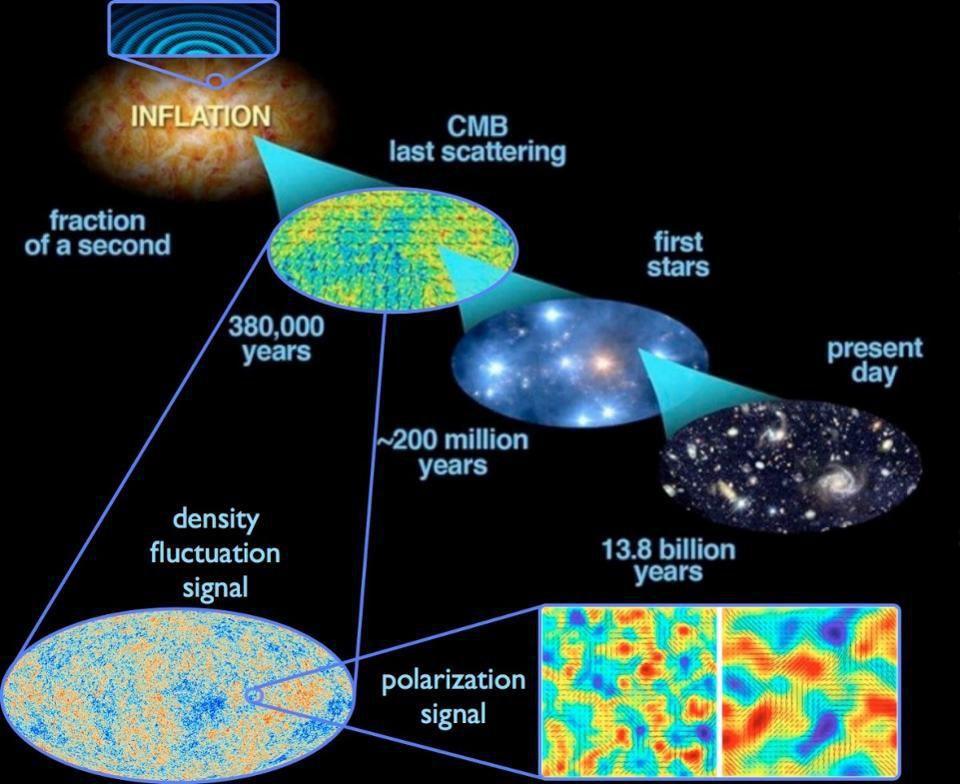
Whereas the original hot Big Bang demanded a singularity, however, the situation now becomes a lot murkier with cosmic inflation added to the mix. Whereas an expanding Universe filled with matter-and-radiation can be traced back to a singularity, in the case of an expanding Universe that’s dominated by some sort of vacuum energy — which is the case for cosmic inflation — the question of a beginning is much less clear.
Because an inflationary spacetime expands exponentially, it can’t be traced back to a singularity; only back to a progressively smaller and smaller — but still finite and non-zero — size.
Whereas a non-inflationary expanding Universe (the classical Big Bang scenario) will allow you to trace every quantum of matter or energy back so that they meet at a single point in the past (the singularity), some geodesics go back an infinite amount in inflationary spacetimes, while others pathologically blow up and/or result in curvature singularities, indicating that inflationary spacetimes, even though they go back eternally, are what’s called “past-timelike incomplete.” This suggests that something very likely preceded cosmic inflation, and although it’s the subject of a lot of interesting ongoing research, the jury is still out on whether those spacetimes must include a singularity or not.
In other words, inflation probably wasn’t “chapter 1” of our Universe’s story either, and it is not presently 100% established whether our Universe began from a singularity or not.
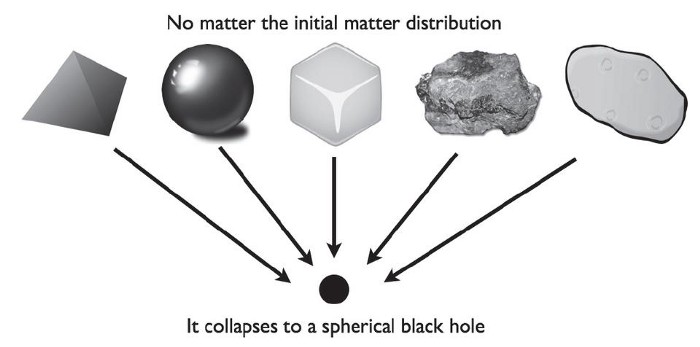
Black holes and their “inevitable” singularities
On the other hand, the situation is very different when it comes to black holes. In fact, it was Einstein himself who first noted that if you took any initial configuration of mass that started off at rest (what relativists idealize as “pressureless dust“) within an otherwise static spacetime, it must inevitably collapse. Not “collapse and form a dust cloud,” but collapse all the way down until it became point-like: until it formed what’s known as a Schwarzschild (non-rotating) black hole.
In the case of a spacetime that contains Schwarzschild black hole, what happens is that far away from the black hole itself, it behaves as any other mass would: deforming and distorting the fabric of spacetime, causing it to curve from its presence, the same way that any other equivalently-valued mass (whether a gas cloud, a planet, star, white dwarf, or neutron star) would deform it.
But unlike those other cases, where the mass is distributed over a large volume of spacetime, in the case of a Schwarzschild black hole, all of that mass collapses down to a single point: a singularity. Around that singularity exists an invisible boundary — a mathematical surface — known as an event horizon, which itself marks the dividing line between where an object, even one moving at the speed of light, can or cannot escape from the gravitational pull of this “hole” in spacetime.

And calling it a “hole” really is appropriate in this instance. In general relativity, we often consider the behavior of what are known as “test particles,” which is to say, something that we can drop down with any property we dream up [mass (including massless), charge, spin, position and speed (including, for massless particles, the speed of light) and a direction for that speed], and ask how it evolves/behaves in the presence of this spacetime. If you want to know what happens within your spacetime — and whether you have a singularity or not, and whether your spacetime is timelike-complete in either the future or past — dropping a series of test particles, including massless ones, is one great way to find out.
In the Schwarzschild spacetime, you can have stable orbits well beyond the vicinity of the event horizon just as you can have planets orbit the Sun or stars move around a galaxy. However, if you get too close to the event horizon, that’s no longer the case. Any quantum of anything that crosses over the event horizon, regardless of its other properties, gets inevitably drawn into the central singularity in a finite (and brief) amount of time. There are no paths around this fate, and nothing that can save you from it.
In fact, the greatest contribution of famed Nobel Laureate Roger Penrose to physics, and in fact the contribution that earned him the Nobel Prize, was the demonstration of how realistic matter, from a collapsing star, actually creates an event horizon and results in a spacetime that terminates in a singularity in the future.
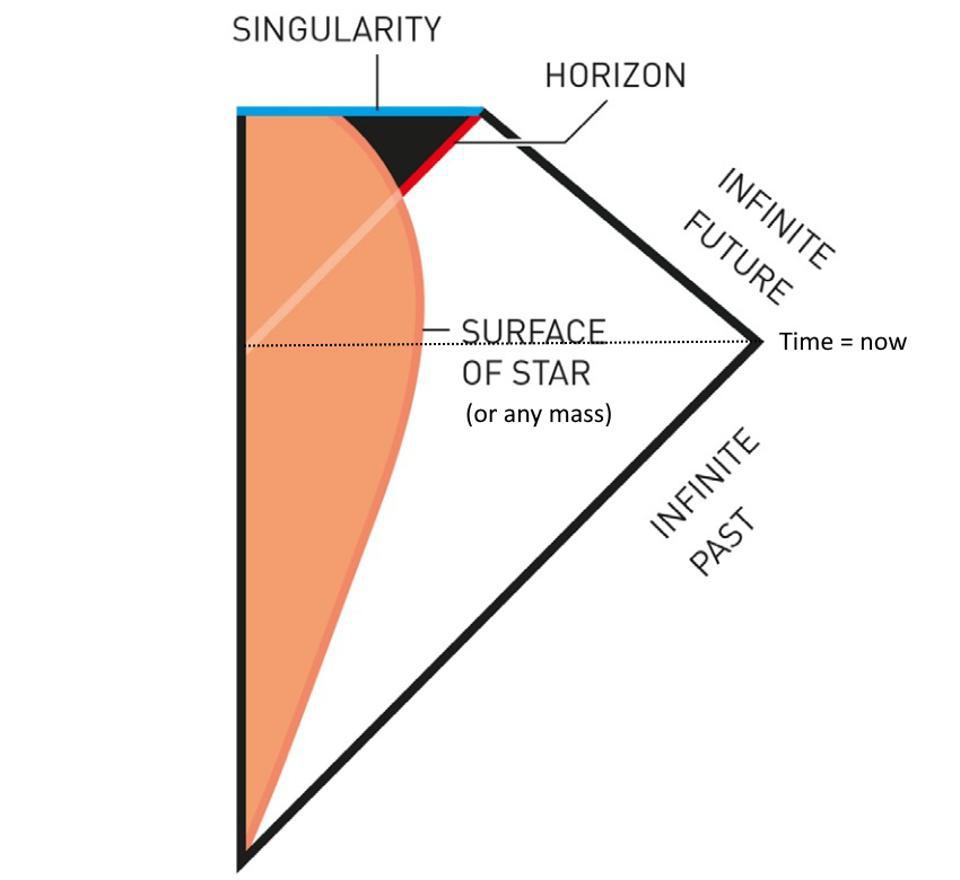
Wiggle room and the chance for a way out
A black hole — even the earliest, simplest conception of a black hole — meets all the necessary criteria for being a spacetime that does, in fact, terminate in a singularity. At that location, there’s a finite, non-zero amount of mass/energy that exists within a single point of infinitesimal size, and that means all the things you’d normally calculate, like density or temperature, would simply blow up and go to infinity. That’s what happens at a singularity, and it truly is a place where pathological behaviors are all that you encounter.
You might try and argue that the Universe, in reality, isn’t described by idealized Schwarzschild black holes. You can instead attempt to add more realistic ingredients, like angular momentum (or spin), and the fact that all of the realistic black holes we’ve observed seem to not only be spinning, but spin at speeds that are quite relativistic, or an appreciable fraction of the speed of light.
And that will get you somewhere: into a different spacetime known as a Kerr spacetime, rather than a Schwarzschild spacetime. A bunch of interesting things happen in this spacetime that don’t occur in the case of non-rotation, including that the event horizon splits in two, into an inner and outer event horizon. There’s also a new in-between region, outside the outer event horizon, known as an ergosphere: where energy and mass can be extracted from just beyond the event horizon.
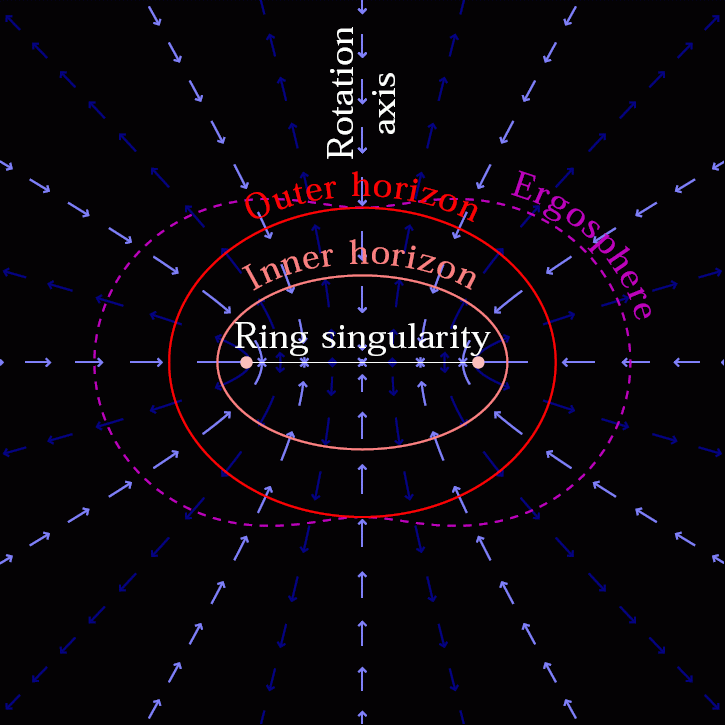
However, there’s still a singularity at the center. While it changes, becoming no longer a point but rather a 1-dimensional object that’s smeared out into a circular ring, it’s still a singularity: a line of infinite density, where again those same pathologies arise, and the laws of physics break down. That attempt to wiggle out won’t get you anywhere.
You can try to imagine that somewhere, inside the event horizon but before you get to the singularity, there’s some compact collection of matter that refuses to collapse further. But that, too, fails due to a fact of Einstein’s relativity: no signal, interaction, or force can move faster than the speed of light. If you wish to have a particle that’s closer to the singularity (from within the event horizon) push back on an outermore particle and keep it from falling in any further, it must propagate back away from the singularity. But all paths from inside the event horizon only lead further down and closer to the central singularity; you’d have to propagate faster than the speed of light to push backward. Unless we throw out relativity altogether, there’s no hope there.
Which leaves only two places left to turn if we want to try and wriggle out of this fate:
- We can appeal to a yet-undiscovered theory that unifies gravitation and quantum theory, like a quantum theory of gravity, and hope that somewhere down the line it allows us to make sensible calculations for what happens where, today, we can only place a singularity.
- Or we can follow the highly speculative (but at least mathematically plausible) idea that perhaps a black hole is actually a gateway to a newborn, baby Universe that exists within it.
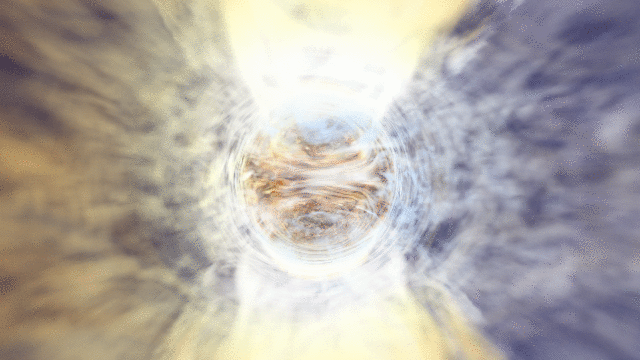
There are many good reasons to hold out hope for the second one, as there’s an interesting mathematical mapping between:
- the inside of a rotating, Kerr black hole as you fall in past the outer event horizon,
- and a spacetime that looks like it expands exponentially, as though it were powered by some sort of energy inherent to the fabric of space itself.
In other words, it’s possible that any infalling material into a realistic black hole will, in some sense (after being ripped apart due to tidal forces and converted into a soup of fundamental quanta), emerge once again into what it perceives as a new Universe, and might potentially experience a hot Big Bang and the resultant cosmic evolution all over again.
However, those are our only two realistic and best hopes for avoiding encountering a central singularity within every black hole. Either quantum gravity will save us (and good luck figuring that one out, as it’s perhaps the most difficult “holy grail” problem in all of theoretical physics), or there’s the possibility that falling into a black hole will chew you up and spit your remnants out in a newborn Universe on the other side. Either way, as long as we’re stuck in our Universe, and as long as the laws of general relativity hold, it appears that a singularity at the center of each black hole really is inevitable.
Send in your Ask Ethan questions to startswithabang at gmail dot com!