The Sun’s Littlest Secret
What makes the Sun shine? For decades, the science didn’t add up.
“Every time we get slapped down, we can say, ‘Thank you, Mother Nature,’ because it means we’re about to learn something important.” –John Bahcall
When you look up at the life-giving ball of fiery plasma in the sky, you might wonder about what it is, exactly, that powers the Sun.
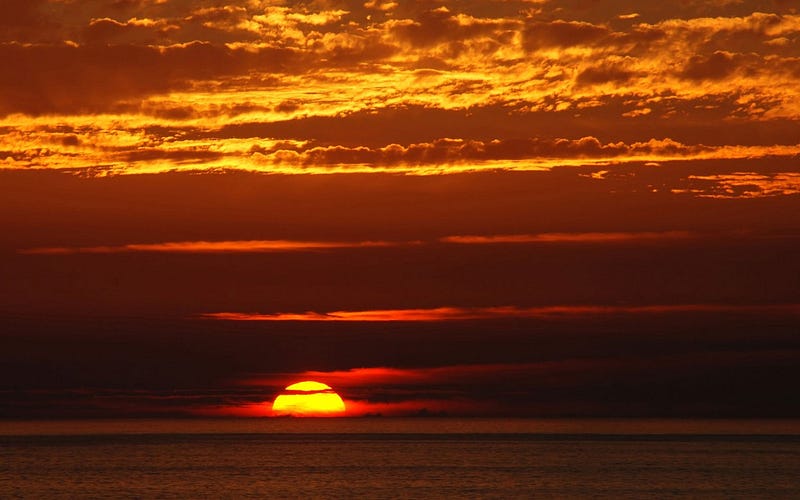
In the late 19th century, the only fundamental forces we knew about were gravitation and electromagnetism, with any understanding of the nuclear forces still lacking. The phenomenon of radioactivity and nuclear transmutation was just being discovered, and so explanations of why the Sun shone so powerfully and for such long time periods relied on wholly insufficient assumptions.
The best estimate for the lifetime of the Sun, as it were, came from Lord Kelvin, who reasoned that the only force capable of emitting such huge energies over large time periods was the force of gravity. Gravitational contraction, he argued, could provide a huge power output over timescales on the order of ten million years. But as any biologist or geologist knew, that was a woefully insufficient (and low) estimate for the age of the features like life or rocks that were plentiful on Earth, and surely the Sun was at least as old as that!

There are objects in this Universe powered by the Kelvin-Helmholtz mechanism, releasing energy via gravitational contraction: white dwarf stars. But these are not representative of the star at the heart of our Solar System.
It wasn’t until the twentieth century, and the discovery that mass can be converted into energy via processes like nuclear reactions, that we had a suitable explanation for why the Sun (and stars) burned with such intense brightness for so long. Through the process of nuclear fusion, light elements (like hydrogen) were getting converted into heavier elements (like helium), releasing a tremendous amount of energy in the process!
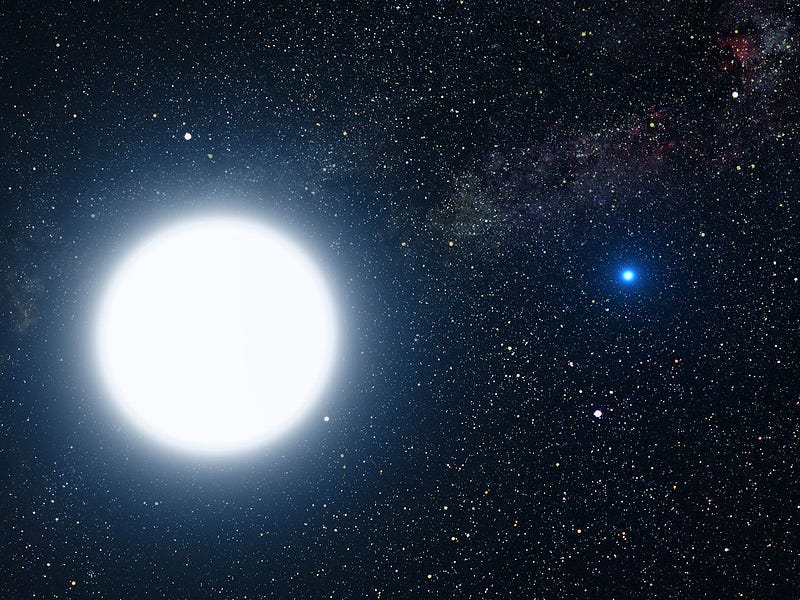
Over the course of its 4.5 billion year lifetime, the Sun has turned about the mass of Saturn into pure energy via E = mc^2, through the conversion of nearly 10^29 kg of hydrogen into helium over this time. Although it was a difficult process, we thought we had figured out the nuclear physics of how this works.
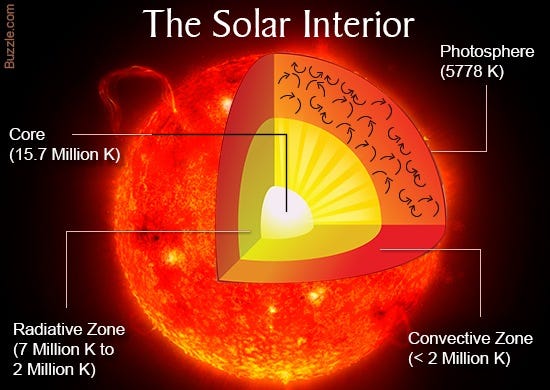
At temperatures above about 4 million Kelvin, all the atoms are ionized, and the energies are high enough that two protons in the core of a star can overcome their mutual electrostatic repulsion so that they get close enough to have the potential to fuse together. This happens thanks to quantum mechanics: their wavefunctions can overlap just enough so that there’s a non-zero chance they’ll wind up becoming bound into a heavier state. That would be deuterium, which is made up of a proton and a neutron bound together.
Deuterium turns out to be a fair bit lighter than two hydrogens, but also requires the production of two other particles: a positron, to conserve electric charge, and an electron neutrino, to conserve lepton number.

Deuterium can then be fused together in a chain reaction to create helium-3 and then helium-4, the isotope of helium most commonly found on Earth (and in stars). All in all, four hydrogen atoms fuse to produce one helium atom, two positrons, and two electron neutrinos. While the energy released by fusion reactions via E = mc^2 — as well as the positrons, which annihilate with electrons to produce even more high-energy photons — is what powers the star, the neutrinos themselves simply escape from the Sun. And some of them make their way towards Earth.
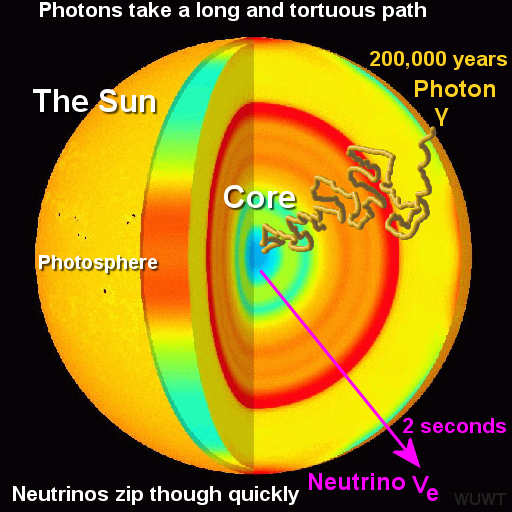
This is where the trouble begins. You see, in the 1950s, we had first detected neutrinos (and their antimatter counterparts, antineutrinos) from nuclear reactors.

When it became clear that the neutrino did exist, and that it was carrying substantial amounts of energy from its creation, we learned two important things:
- its cross-section, or how frequently it’d interact with normal matter, was both energy dependent and extremely small, but measurable, and
- that if we built a detector for them and knew their flux and their energy, we should be able to accurately predict the interaction rate.
It seemed like the perfect storm! We knew the physics of the Sun, and how these nuclear reactions took place. We knew about neutrinos, what their cross-section was, and how the cross-section behaved as a function of energy. And we even believed we had a good model — thanks to people like the aforementioned John Bahcall — of the interior of the Sun and with what properties it produced neutrinos.
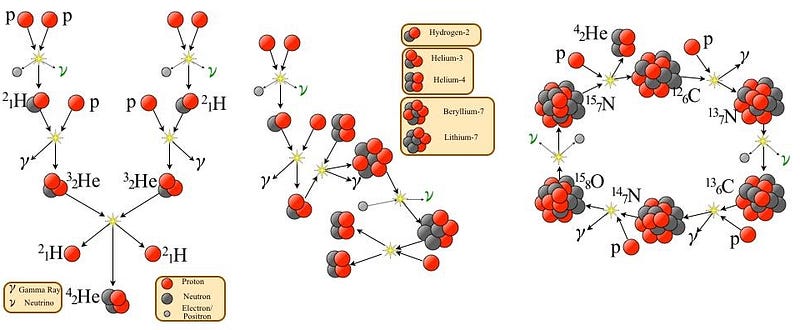
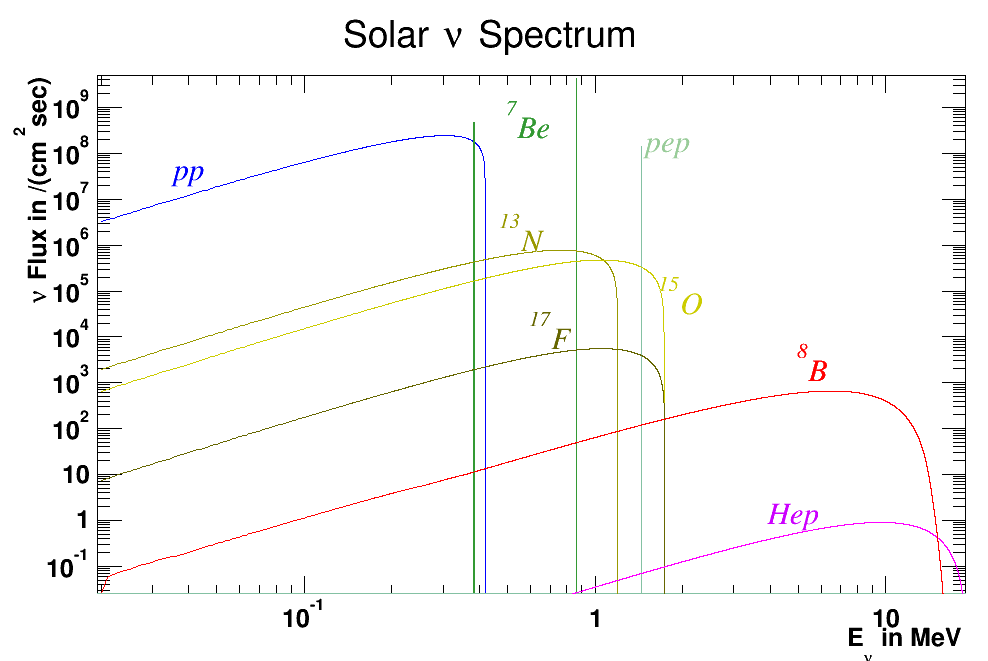
Which is why it was such a puzzle when, in the 1960s, the first measurements of the neutrino flux from the Sun was made, and it turned out to be only a third of what we anticipated it would be. Many, many wild speculations abounded, including some incredibly reasonable ideas:
- Perhaps the models of the Sun’s interior were wrong, and the neutrino fluxes were occurring at different energies than what we were seeking.
- Perhaps our understanding of neutrino detection — and how that cross-section scaled with energy — was different from what the reality was.
- Or, perhaps, there was some new physics going on as far as neutrinos were concerned.
As someone with a well-deserved reputation for almost always taking the conservative approach when it comes to new physics, I would’ve certainly bet on one of the first two possibilities.
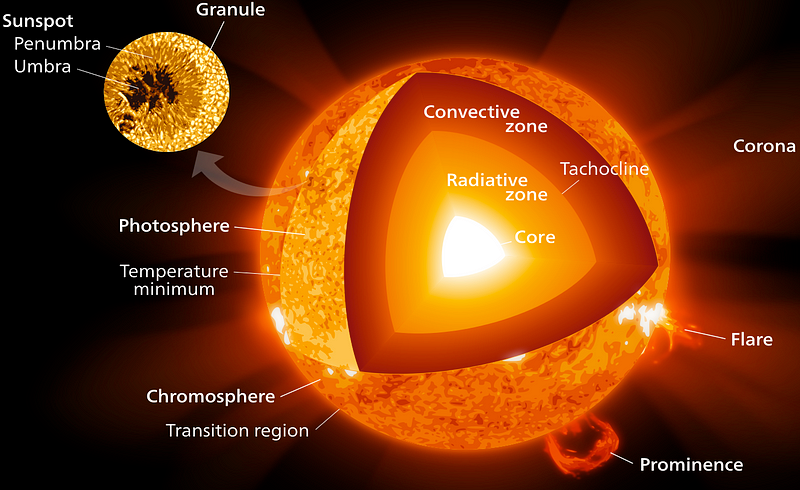
And yet, as our understanding of ultra-high-temperature physics improved, as our understanding of stars and the Sun in particular improved, and as our understanding of neutrinos, their properties, and their detection improved, it really began to appear that it would require some new physics to solve this problem. As we started to build incredibly large neutrino observatories, that same problem — that only a third of the neutrinos from the Sun were arriving at our detectors — persisted.
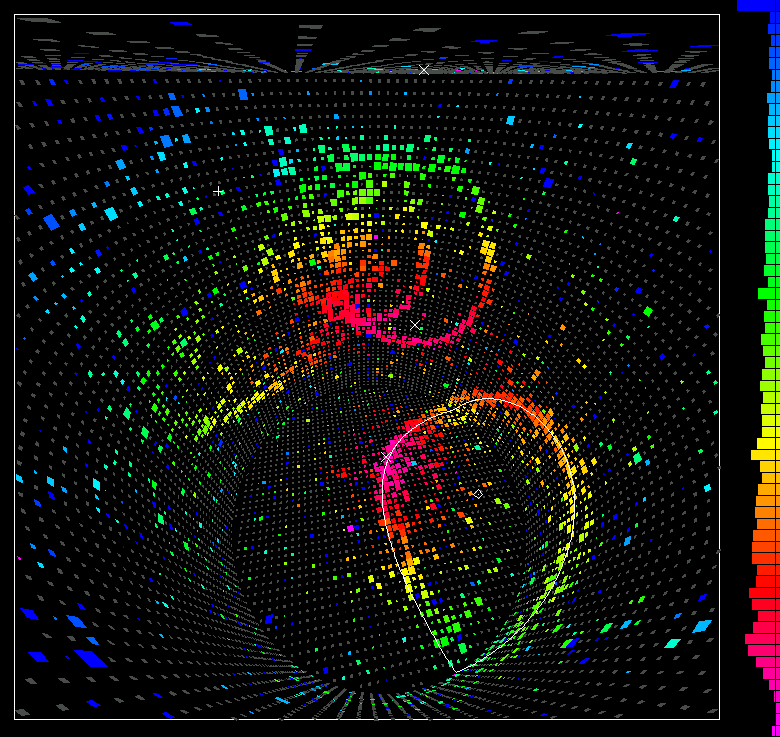
Neutrinos, you see, are among the most weakly interacting particles of all in the standard model. They’re stable, they only interact through the weak force, they have no electric charge and do not scatter off of light. And, for a very long time, they were thought to have zero mass.
But if you take a look at the standard model, you’ll find that there isn’t just one neutrino.
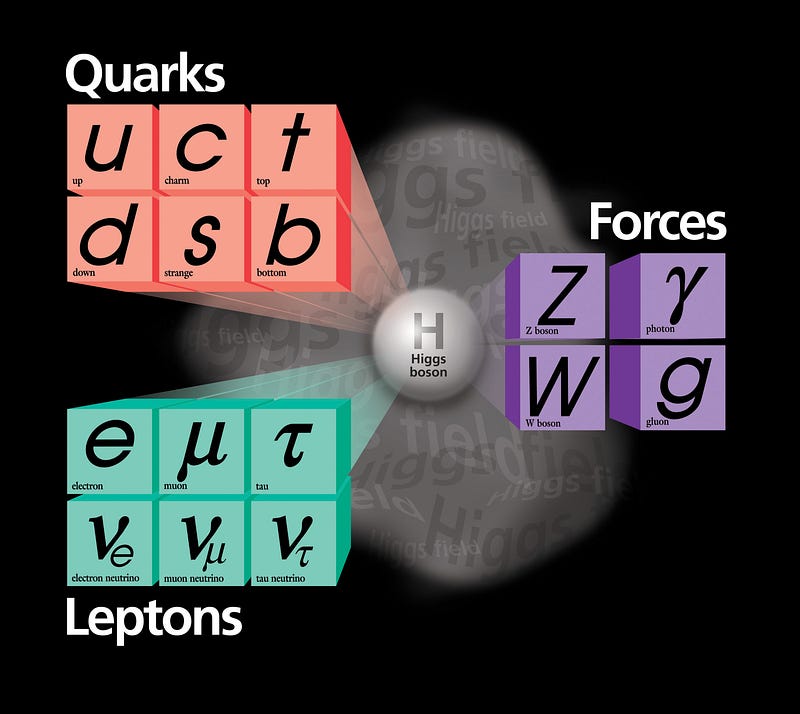
Just as there are three types of charged lepton: the electron, muon and tau, there are three types of neutrino as well: the electron neutrino, muon neutrino and tau neutrino. If the neutrinos were absolutely distinct from one another and completely massless, then if you were born an electron neutrino, you’d die an electron neutrino, and never would you ever become anything else.
But if the neutrinos had mass to them, it was possible that they could interact with the intervening matter in the Sun — the electrons in particular — to change flavor, from electron to muon to tau and back again.
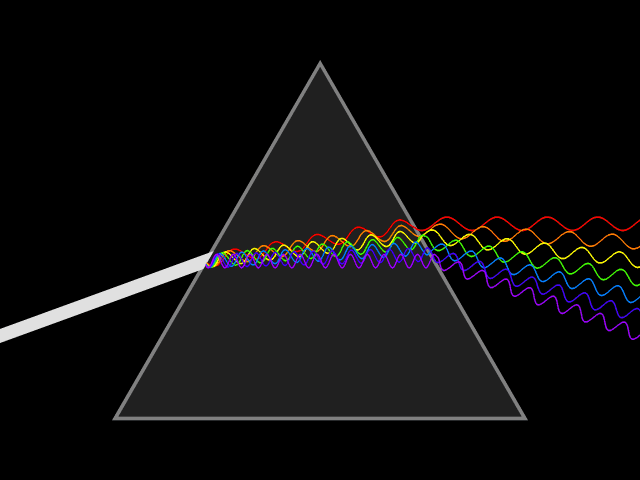
Just like light refracts when you pass it through a medium, bending both dependent on its wavelength and on the different speed-of-light in that medium, neutrinos in a medium behave as though they have different masses dependent on the density of that medium. Since the Sun has a rapidly changing electron density as you exit it from its core, this effect, known as the Mikheyev-Smirnov-Wolfenstein effect, causes a flavor-changing of the neutrinos. While they all started out as electron neutrinos in the Sun’s interior, by time they get to the photosphere, they’re well-mixed, with about a third of them electron-neutrinos, a third muon-neutrinos and a third tau-neutrinos.
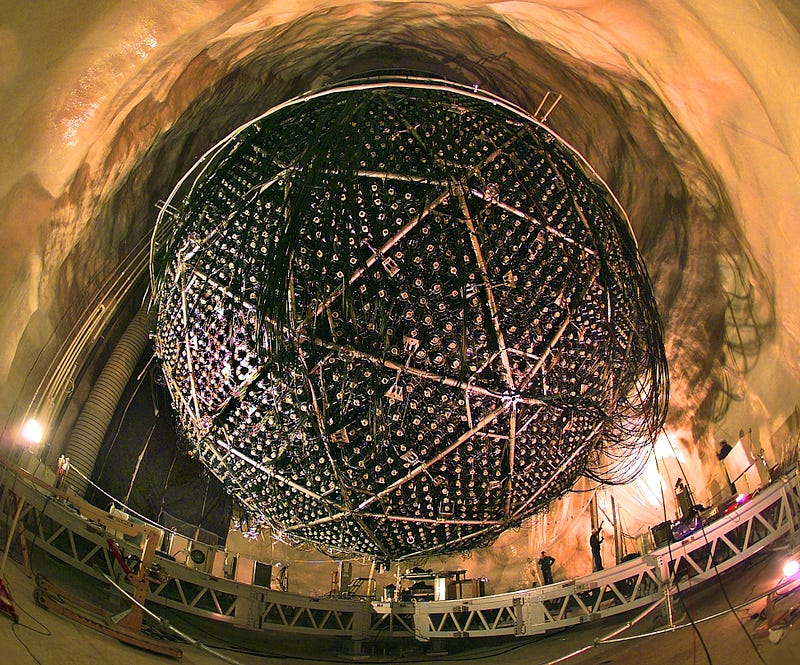
It wasn’t until the early 2000s when the Sudbury Neutrino Observatory, above, was able to measure the total neutrino flux from the Sun — via a scattering effect — and simultaneously also the electron neutrino flux from the Sun, and determine that 34% of the neutrinos were electron neutrinos, with the other two thirds being split among the other two types. Subsequently, measurements of atmospheric neutrinos have taught us even more about neutrino oscillation, and the ability of these elusive particles to transform from one type into another as they travel through space is one of the most compelling hints about what new physics might lie beyond the Standard Model.
At last, John Bahcall was vindicated! His models of the Sun were correct, and so were his predictions about what the cause of this discrepancy was: it was the neutrinos’ fault, after all, and there was new physics afoot!
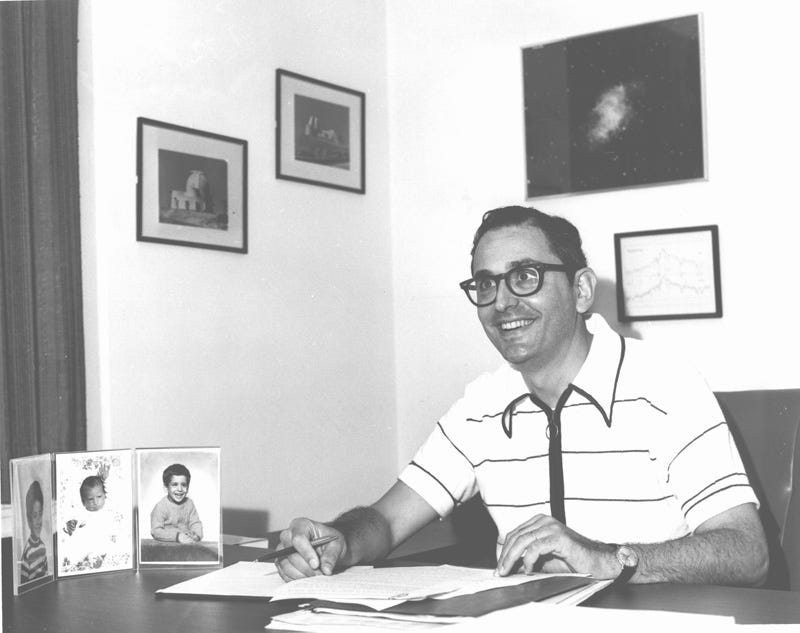
John Bahcall died in 2005 of a rare blood disorder, but lived to see his model of the Sun and the theory of neutrino oscillation confirmed. I was lucky enough to see him speak on the topic just a little over a year before his death, and I think he’d be very pleased today to know all that we’ve learned about the small but non-zero masses of neutrinos, their importance for cosmology and astrophysics, the completion of the Standard Model and where we currently stand in our search for the underlying physics behind neutrino oscillation.
Why do neutrinos have mass? What mass do they have, exactly? And what other new, fundamental particles exist that enables all of this? These are some of the new holy grail questions: the questions that will take particle physics truly into the third millennium, and — at last — beyond the standard model.
Leave your comments at the Starts With A Bang forum on Scienceblogs!