Why Neutron Stars, Not Black Holes, Show The Future Of Gravitational Wave Astronomy
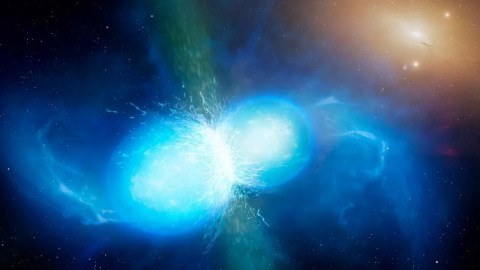
The first detections were incredible. But now the real fun — and the real science — truly begins.
On August 17, the signals from two merging neutron stars reached Earth after a journey of 130 million light years. After an 11 billion year dance, these remnants of once-massive, blue stars that died in supernovae so long ago spiraled into one another after emitting enough gravitational radiation to see their orbits decay. As each one moves through the changing spacetime created by the gravitational field and motion of the other, its momentum changes, causing the two masses to orbit one another more closely over time. Eventually, they meet, and when they do, they undergo a catastrophic reaction: a kilonova. For the first time, we’ve recorded the inspiral and merger in the gravitational wave sky, noticing it in all three detectors (LIGO Livingston, LIGO Hanford, and Virgo), as well as in the electromagnetic sky, from gamma rays all the way through the optical and into the radio. At last, gravitational wave astronomy is now a part of astronomy.
We knew this had to happen eventually. Neutron stars have very large masses, estimated at over the mass of the Sun each, and very small sizes. Imagine an atomic nucleus that didn’t contain a handful, a few dozen, or even a few hundred protons and neutrons inside, but rather a star’s worth: 1057 of them. These incredible objects swoop through space, faster and faster, as the fabric of space itself bends and radiates due to their mutual presence. Pulsars in binary systems coalesce, and in the very final stages of inspiral, the strain they impose on a detector even a hundred million light years away can be detectable. We’ve seen the indirect evidence for decades: the decay of their mutual orbits. But the direct evidence, now available, changes everything.
Each time these waves pass through your detector, they cause a slight expanding-and-contracting of the laser arms. Because the neutron star system is so thoroughly predictable, decaying at the rate predicted by Einstein’s equations, we know exactly how the frequency and amplitude of the inspiral ought to behave. Unlike black hole systems of higher masses, the frequency of these low-mass systems falls in the detectable range of the LIGO and Virgo detectors for much longer time periods. While the overwhelming majority of black hole-black hole mergers registered in the LIGO detectors for only a fraction of a second, these neutron stars, even at a distance of over 100 million light years, had their signals detected for almost half a minute!
This time, the Fermi gamma-ray satellite detected a transient burst, consistent with previously seen kilonovae, just 1.7 seconds after the arrival of the final “chirp” of the gravitational wave signal. By time 11 hours had passed, the LIGO/Virgo team had pinpointed an area on the sky just 28 square degrees in size: the smallest localized region ever seen. Even though the neutron star signal was so much less intense in magnitude than the black hole signals were, the fact that the detectors had caught so many orbits gave the team the strongest signal to date: a signal-to-noise ratio of more than 32!
By knowing where this signal was, we could then train our greatest optical, infrared, and radio telescopes on this site in the sky, where the galaxy NGC 4993 was located (at the correct distance). Over the next two weeks, we saw an electromagnetic counterpart to the gravitational wave source, and the afterglow of the gamma-ray burst that Fermi saw. For the first time, we had observed a neutron star merger in gravitational waves and across the light spectrum, confirming what theorists had suspected in spectacular fashion: that this is where the majority of the heaviest elements in the Universe originate.
But also encoded in this merger are a few incredible facts that you may not realize; facts that point the way to the future of gravitational wave astronomy.
1.) Binary neutron stars barely spin at all! In isolation, neutron stars can be some of the most rapidly spinning objects in the Universe, up to a significant percentage of the speed of light. The fastest rotate over 700 times per second… but not in a binary system! The close presence of another large mass means that tidal forces are large, and hence the friction of one rotating body on another causes them both to slow down. By time they merge, neither one can be rotating at any appreciable speed, allowing us to constrain the orbital parameters from the gravitational wave signal extremely tightly.
2.) At least 28 Jupiter masses’ worth of material was converted into energy via E = mc². We’ve never seen neutron star-neutron star mergers in gravitational waves before. In black hole-black hole systems of equivalent mass, up to 5% of the total mass gets converted into energy. In neutron star systems, its expected to be less, because the collision occurs between nuclei, not between singularities; the two masses can’t get as close. Still, at least 1% of the total mass was converted into pure energy via Einstein’s mass-energy equivalence, a very impressive and large amount of energy!
3.) Gravitational waves move at exactly the speed of light! Before this detection, we never had a gravitational wave and a light signal simultaneously identifiable to compare with one another. After a journey of 130 million light years, the first electromagnetic signal from this detection arrived just 1.7 seconds after the peak of the gravitational wave signal. That means, at most, the difference between the speed of gravity and the speed of light is about 0.12 microns-per-second, or 0.00000000000004%. It’s anticipated that these two speeds are exactly equal, and the delay of the light signal comes from the fact that the light-producing reactions in the neutron star take a second or two to reach the surface.
4.) A faster response time is possible! By time we first located the three-dimensional place on the sky where the electromagnetic signal was, twelve hours had passed. Sure, we were able to observe the optical counterpart immediately, but it would’ve been better to get in on the ground floor. As automated analysis improves, as well as the synchronization of all three detectors, the better we’ll do. Over the coming years, LIGO will get slightly more sensitive, Virgo will do better, and two additional LIGO-like detectors, KAGRA in Japan and LIGO-India, will come online. Instead of half a day, we may be soon talking about response times in a matter of minutes or even seconds.
5.) Going to space will be the ultimate in gravitational wave observing. Here on the ground, part of the reason it took so long to find the location was that in Livingston, LA, there was a “noise” glitch: something caused the detector on the ground to vibrate. As a result, the automated software couldn’t extract the true signal, and manual intervention was required. The LIGO-Virgo team did an amazing job, but were these detectors in space, this wouldn’t even have been an issue in the first place. There is no seismic noise in the abyss of interplanetary space.
Unlike merging black holes, inspiraling and merging neutron stars:
- Can be seen for a much longer time, due to their low masses,
- Will emit electromagnetic counterparts, allowing for the gravitational and electromagnetic skies to be unified,
- Are far more numerous, with the only reason we’ve seen more black holes is due to the increased range for them,
- And can be used to learn information about the Universe, such as the speed of gravity, that black holes cannot teach us.
The delay of around 11 hours from the merger to the first optical and infrared signatures isn’t due to physics, but due to our own instrumental limitations here. As our analysis techniques improve, and more events are discovered, we’ll learn exactly how long it takes before visible light signatures are created by neutron star-neutron star mergers.
At last, the origin of the heavy elements are confirmed; the speed of gravity is definitively known; and the gravitational wave and electromagnetic skies are one. Any doubters of LIGO now have the independent confirmation they’ve been clamoring for, and there is no ambiguity left. The future of astronomy includes gravitational waves, and that future is here, today. Congratulations, one and all. Today, all of Earth is the beneficiary of this incredible knowledge.
Ethan Siegel is the author of Beyond the Galaxy and Treknology. You can pre-order his third book, currently in development: the Encyclopaedia Cosmologica.