Ask Ethan: Did The LHC Discover A New Type Of Particle?
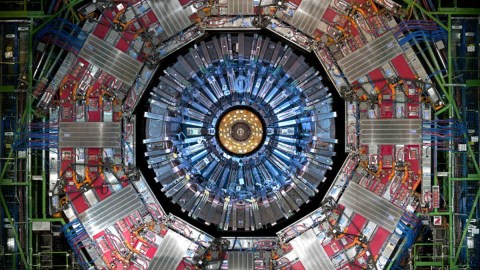
And what, exactly, is the significance of a tetraquark?
“I found I could say things with color and shapes that I couldn’t say any other way — things I had no words for.” –Georgia O’Keeffe
In the quest to advance our knowledge of the Universe, the biggest advances always seem to come when an experiment or measurement indicates something new: something our best theories to that date hadn’t predicted before. We all know that the LHC is looking for fundamental particles beyond the Standard Model, including hints of supersymmetry, technicolor, extra dimensions and more. Is it possible that the LHC just discovered a new type of particle, and the results just got buried in the news? That’s the question of Andrea Lelli, who wants to know why
The news about tetraquark particles discovered in the LHC was published in some scientific feeds, but it seems the news did not catch mainstream attention. Isn’t this a valuable discovery, even though tetraquarks were already theorized? What does it mean for the standard model exactly?
Let’s find out.
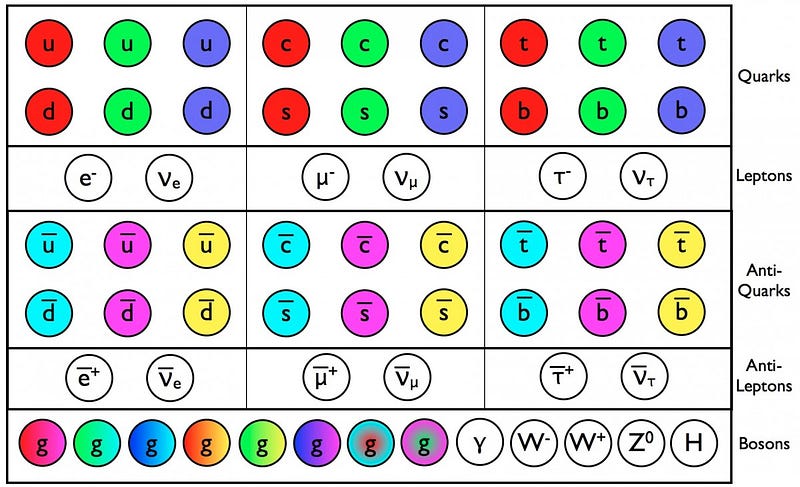
When it comes to the particles we know in the Universe, we have:
• the quarks, which make up protons and neutrons (among other things)
• the leptons, including the electron and the very light neutrinos,
• the antiquarks and antileptons, the antiparticle counterparts of the above two classes,
• we have the photon, the particle version of what we call light,
• we have the gluons, which bind the quarks together and are responsible for the strong nuclear force,
• we have the heavy gauge bosons — the W+, W- and the Z0 — which mediate the weak interactions and radioactive decays,
• and the Higgs boson.
The main goal of the LHC was to find the Higgs, which it did, completing the gamut of expected particles in the Standard Model. The stretch goal, however, was to find new particles beyond the ones we expected. We hopes to find clues to the greatest unsolved problems in theoretical physics at these high energies. To find something that could provide a hint to dark matter, the matter-antimatter asymmetry of the Universe, the reason particles have the masses they do, the reason strong decays don’t occur in certain fashions, etc. To find a new fundamental particle, and to give us either experimental support for a speculative theoretical idea or to surprise us, and push us in a new direction entirely.
The closest we’ve gotten to that is a “hint” of a new particle whose decay shows up in the two-photon channel at 750 GeV. The threshold for discovery, however, requires a significance indicating there’s less than a 0.00003% chance of a fluke; the CMS and ATLAS data are at a 3% and a 10% chance of a fluke, respectively. That’s a pretty tenuous hint.
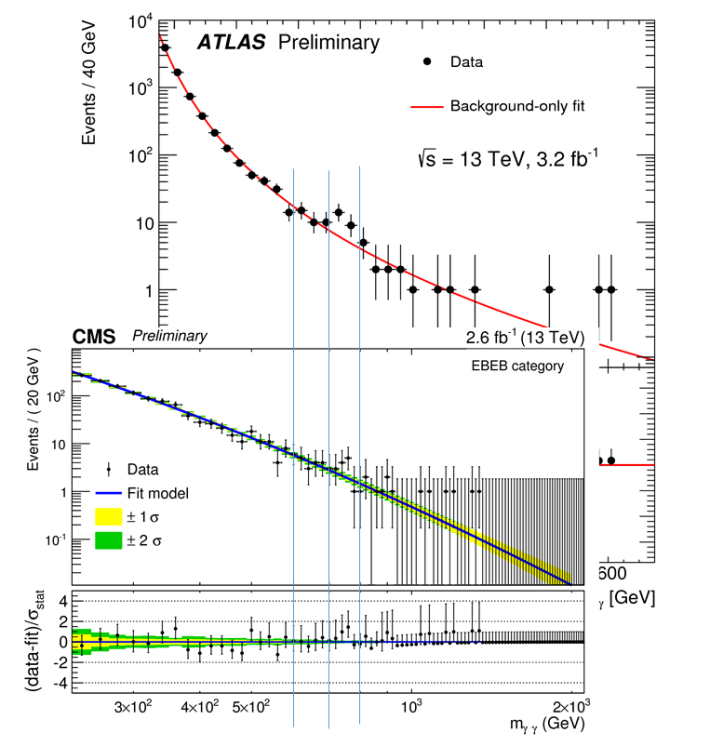
But the LHC does have a few new discoveries under its belt, although they aren’t quite fundamental discoveries in the “new particle” sense. What we got instead, however, was an announcement about the discovery of tetraquarks. These aren’t new particles that are additions or extensions to the standard model: they don’t represent new forces, new interactions, or potential solutions to any of the great, outstanding problems of theoretical physics today. Rather, they’re entirely combinations of the existing particles that have never been seen before.
The way quarks work is they come with a color: red, green or blue. (Antiquarks are cyan, magenta and yellow, respectively: the anti-colors of the quarks.) Gluons are exchanged between quarks to mediate the strong nuclear force, and they change the quark (or antiquark) colors when they do. But here’s the kicker: in order to exist in nature, any combination of quarks or antiquarks must be completely colorless. So you can have:
• Three quarks, since red+green+blue = colorless.
• Three antiquarks, since cyan+magenta+yellow = colorless.
• Or a quark-antiquark combination, since red+cyan (i.e., anti-red) = colorless.
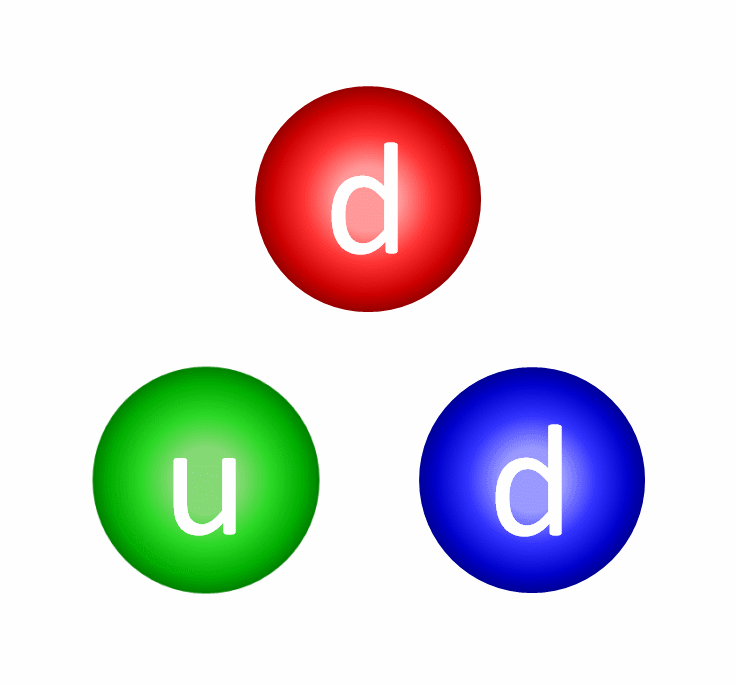
(You can also think of colors as “arrow” vectors in particular directions, and you have to get back to the origin to make something colorless.)
The three quark combinations are known as baryons, and protons and neutrons are two such examples, along with more exotic combinations involving heavier quarks. Combinations of three antiquarks are known as anti-baryons, and include anti-protons and anti-neutrons. And the quark-antiquark combinations are known as mesons, which mediate the forces between atomic nuclei and have interesting life-and-decay properties on their own. Meson examples include the pion, the kaon, charmonium and the upsilon.
But why stop there? Why not envision other color-free combinations? Why not something like:
• Two quarks and two antiquarks, a tetraquark?
• Or four quarks and one antiquark, a pentaquark?
• Or even something like five quarks and two antiquarks, a heptaquark?

(Having six quarks isn’t interesting or new: we already know how to make deuterium, a heavy isotope of hydrogen.) According to the Standard Model, this is not only possible, this is predicted. It’s a natural consequence of quantum chromodynamics: the science behind the strong nuclear force and those interactions.
In the early 2000s, it was claimed that pentaquarks — these five quark/antiquark combinations — were discovered. Unfortunately, this was premature, as the 2003 result from Japan’s Laser Electron Photon Experiment at SPring-8 (LEPS) was unable to be reproduced and the other mid-2000s results were of poor significance. Tetraquark states were coming out at right around the same time. In 2003, the Belle experiment (also in Japan) announced a very controversial result: the discovery of a particle with a mass of 3872 MeV/c^2 whose quantum numbers did not match any feasible baryon or meson-like states. For the first time, we had a tetraquark candidate.
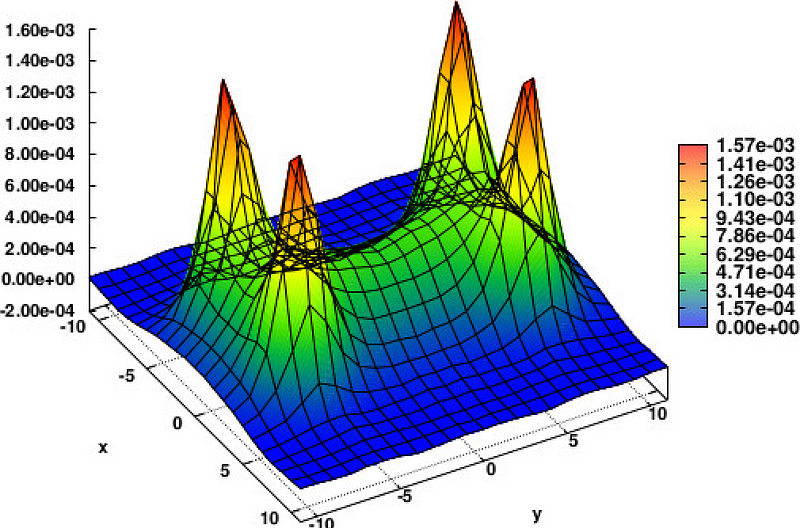
Belle went on, in 2007, to discover two other tetraquark candidates, including the first one with charm quarks inside of it, while Fermilab also uncovered a number of tetraquark candidates. But the biggest breakthrough in these “other” combination states came in 2013, when both Belle and the BES III experiment (in China) independently reported the discovery of the first confirmed tetraquark state. It was the first tetraquark to be directly observed experimentally. Just like pions, it comes in positively charged, negatively charged and also neutral versions.
Since then, the LHC has taken the lead, collecting more data on high-energy hadrons than any other experiment before it. The LHCb experiment, in particular, is the one designed to observe these particles. Some tetraquark candidates — like Fermilab’s bottom-quark containing candidate from the DØ experiment — were disfavored by the LHC. But others were directly observed, like Belle’s 2007 charm-containing tetraquark along with many new ones. And the latest tetraquark results that you allude to, reported here in Symmetry Magazine, detail four new tetraquark particles.
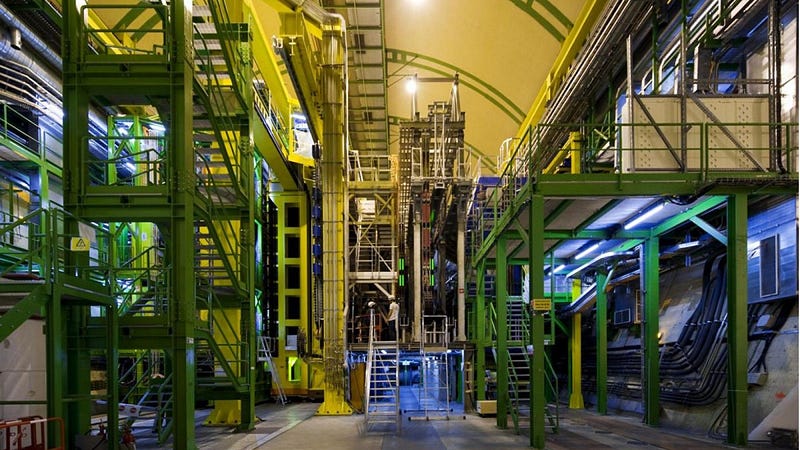
The cool thing about these four new particles is that they’re made up of two charm and two strange quarks apiece (with two always being the “anti” version), making these the first tetraquarks to have no light (up and down) quarks in them. And just like you can have a single electron within an atom exist in many different unique states, the way these quarks are configured means that each of these particles have unique quantum numbers, including mass, spin, parity, and charge conjugation. Physicist Thomas Britton, who did much of this work for his Ph.D., detailed:
We looked at every known particle and process to make sure these four structures couldn’t be explained by any pre-existing physics. It was like baking a six-dimensional cake with 98 ingredients and no recipe — just a picture of a cake.
In other words, we’re 100% positive these aren’t any normal hadrons the Standard Model could have predicted, and pretty sure these really are tetraquarks!
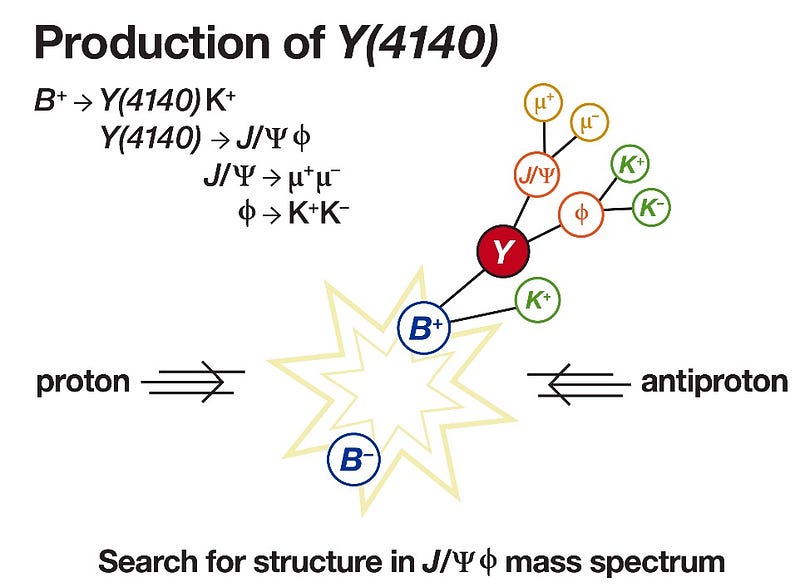
The way they normally show up — as the picture details above — is by showing up in an intermediate stage (indicated by Y) of some decays. This is completely allowed by the Standard Model, but it’s a very rare process and so, in some sense, it’s amazing that we have the sheer amount of data and can measure it precisely enough to detect these classes of particles at all. Tetraquarks, pentaquarks and even higher combinations are expected to be real. Perhaps most oddly of all, the Standard Model predicts the existence of glueballs, which are bound states of gluons.
It’s important to remember that in doing these tests, and in looking for these incredibly rare and difficult-to-find states of nature, we are doing the highest-precision tests of QCD — the theory underlying the strong force — of all-time. If these predicted states of quarks, antiquarks and gluons fail to materialize, then something about QCD is wrong, and that would be a way of going beyond the Standard Model, too! Finding these states is the first step; understanding the details of how they fit together, what their hierarchies are and how our known physics applies to these more and more complex systems is what comes next. As with everything in nature, the payoff for human advancement is hard to see when the initial discovery is made, but the joy of finding things out is always its own reward.
This post first appeared at Forbes, and is brought to you ad-free by our Patreon supporters. Comment on our forum, & buy our first book: Beyond The Galaxy!