Does dark matter interact non-gravitationally at all?
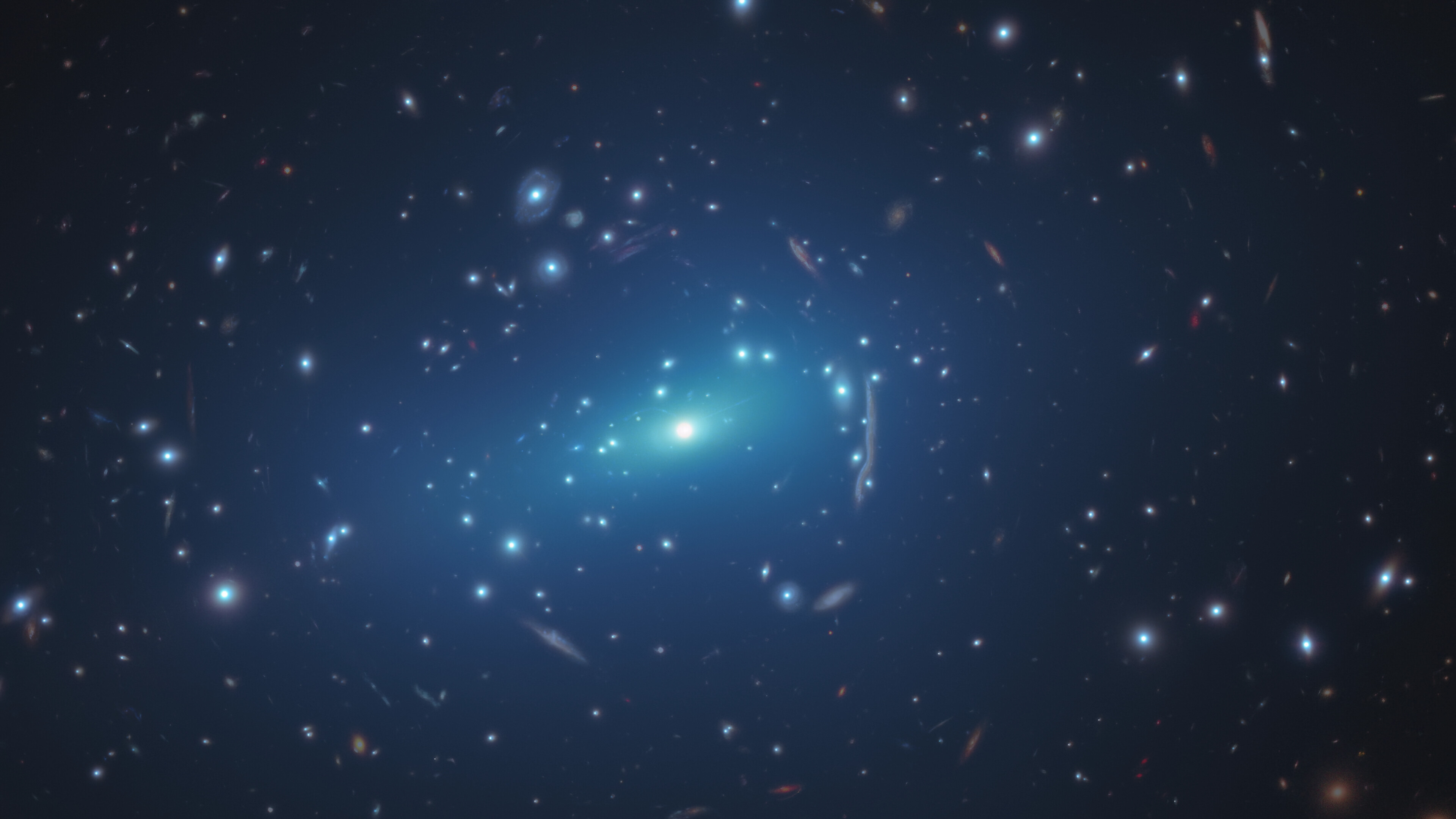
- To explain the gravitational effects that cannot be accounted for by the presence of normal matter alone within general relativity, the presence of dark matter is the leading scientific hypothesis.
- Known to be cold, collisionless, and non-interacting with either itself, light, or normal matter through any force other than gravity, it explains an enormous suite of cosmological observations and solves many puzzles.
- However, certain classes of low-mass galaxies, especially ultra-diffuse galaxies, exhibit vastly different behavior from what “standard” dark matter predicts. Is this evidence for a non-gravitational force?
One of the biggest cosmic puzzles in the entire Universe is dark matter. If we take for granted that we know:
- the laws of physics (the Standard Model plus general relativity),
- the origin of our Universe (the hot Big Bang preceded by a phase of cosmic inflation),
- and the contents of our Universe (the amount of matter comprised of Standard Model particles),
our cosmic picture fails to add up. From how galaxies rotate to how they move within galaxy clusters to gravitational lensing profiles to the total mass/energy of the Universe to how the Universe expands over time to features in the Big Bang’s leftover glow and the large-scale clustering of galaxies on cosmic scales, a Universe with only the Standard Model particles in it fails to match observations. Instead, two additional ingredients — dark matter and dark energy — are usually added to complete the picture, where everything then falls back into line with what’s observed.
As far as dark matter is concerned, this addition is troubling to many. Whereas the cumulative amount of energy in the Universe from Standard Model particles (including atomic ingredients, photons, neutrinos, and more) makes up only around 5% of the total cosmic energy budget, dark matter must make up around 27%: outmassing normal matter by around a 5-to-1 ratio. If we assume that dark matter is cold, collisionless, and interacts only through gravity, it can reproduce almost all of the observations ever collected. However, a sub-population of galaxies — the ultra-diffuse dwarf galaxies — stands in defiance to the conventional paradigm. Do they indicate, as some have asserted, that the standard collisionless cold dark matter picture no longer works? It’s a controversial take, so let’s dive into the science behind this puzzle.
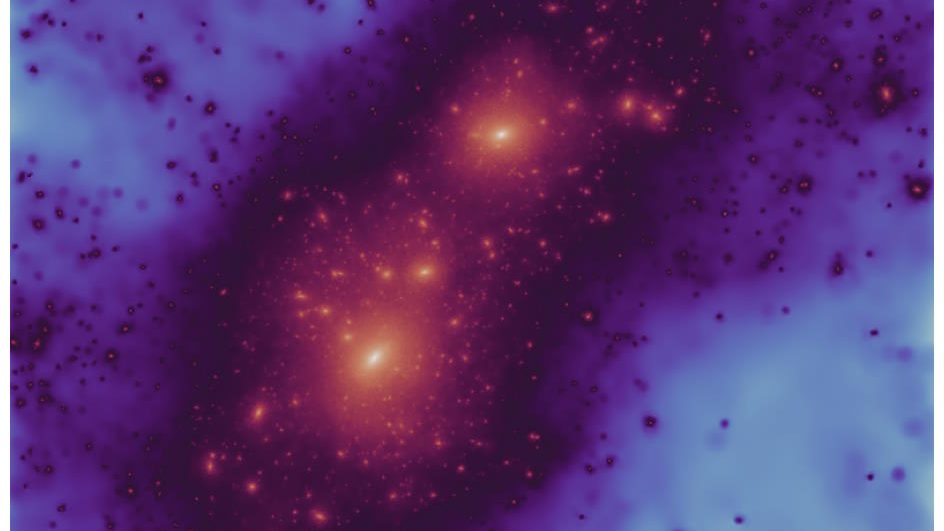
It’s easy to look at the standard picture of our Universe and find yourself dissatisfied with our current perspective on how things work. We have an extraordinary amount of knowledge about the known particles and forces in our Universe: everything in the Standard Model (quarks, leptons, and bosons), which represents all of the normal matter, radiation, and neutrinos and antineutrinos that we know of, as well as the forces of gravity (general relativity) and the three quantum forces (weak nuclear, strong nuclear, and electromagnetic, governed by the Standard Model) that are known to exist.
And yet, if we assume “these are the only forces that exist,” we not only have a slew of unsolved problems to face down, but we have to reckon with the fact that only about ~5% of the total energy in the Universe is described by what is presently known.
It’s certainly a great leap to add another ingredient to the Universe — one whose presence has never been directly detected, but only where evidence arises for it indirectly — much less two more ingredients, but that’s precisely the scenario we have to face with dark matter and dark energy. The reason our “consensus cosmology” includes these two ingredients, as opposed to incorporating either new forces or a modification to the existing forces, is that a whole suite of observations (practically all of them) can be explained by adding dark matter and dark energy, whereas modifying the forces or adding new forces still require us to add new matter ingredients (such as dark matter) as well to bring cosmology into balance.
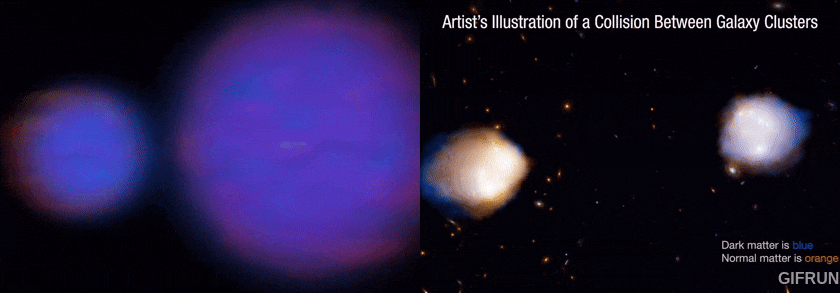
On large cosmic scales, the evidence for dark matter — although indirect — is absolutely overwhelming.
- The pattern of fluctuations in the cosmic microwave background indicate an additional component of matter in the Universe that does not collide with radiation the way that normal matter does, and that the “additional matter” exists in about five times the abundance of normal matter.
- The large-scale clustering of galaxies indicates that there’s an “acoustic scale” to the Universe, where pairs of galaxies are more likely to be found 500 million light-years away from one another than 400 or 600 million light-years away, and that scale (the acoustic scale) evolves as the Universe expands: a feature that indicates the presence of both dark and normal matter, and indicates that same 5-to-1 ratio as well.
- Observations of strong lensing systems, where both individual galaxies and galaxy clusters are the gravitational lenses, indicates that not only are there enormous and diffuse dark matter halos found around these objects, but that they have “clumpy” substructure within them, as predicted by dark matter simulations.
- And, perhaps most spectacularly, galaxy clusters that collide with one another are witnessed to have their gravitational signal “separate” from the signature of most of the normal matter: X-ray emitting gas.
This last observation, first made in 2005 (long after the dark matter paradigm had been established), is often hailed as an empirical proof of dark matter’s existence, as no dark matter-free theory can account for these observations in a compelling fashion.
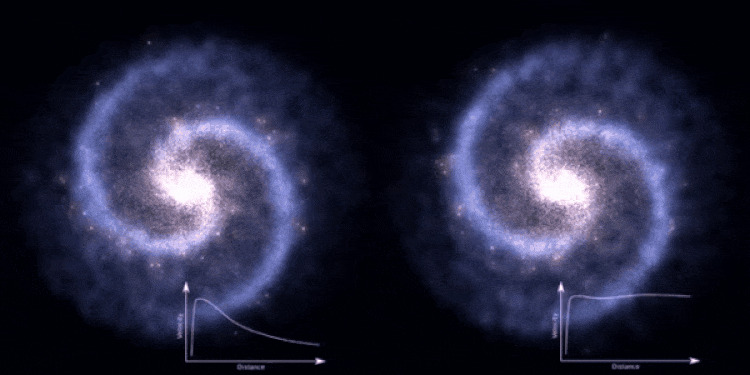
However, there’s still the problem of individual galaxies. If we assume that dark matter is truly cold and collisionless, and never interacts with normal matter or radiation or any Standard Model particle in any way (other than gravitationally), then simulations indicate that dark matter should obey what we call a universal profile. In a universal profile, under the influence of gravity, dark matter particles will come to distribute themselves in a halo-like structure, with densities that:
- rise as you come inward toward the center,
- then “turn over” to rise at a lesser rate,
- and that make a “cusp” in density, which peaks at the very center itself.
Originally a product of simulations of dark matter alone dating back to 1995, when Julio Navarro, Carlos Frenck, and Simon White calculated it, this predicted “universal profile” helps explain why large spiral and elliptical galaxies have flattish rotation curves, but often fails to predict the specific behavior of individual galaxies in gory detail. Although the broad strokes are correct, there is no “cusp” seen at the centers of most galaxies due to dark matter, and the inferred density distributions do not match what the universal profile for dark matter predicts.
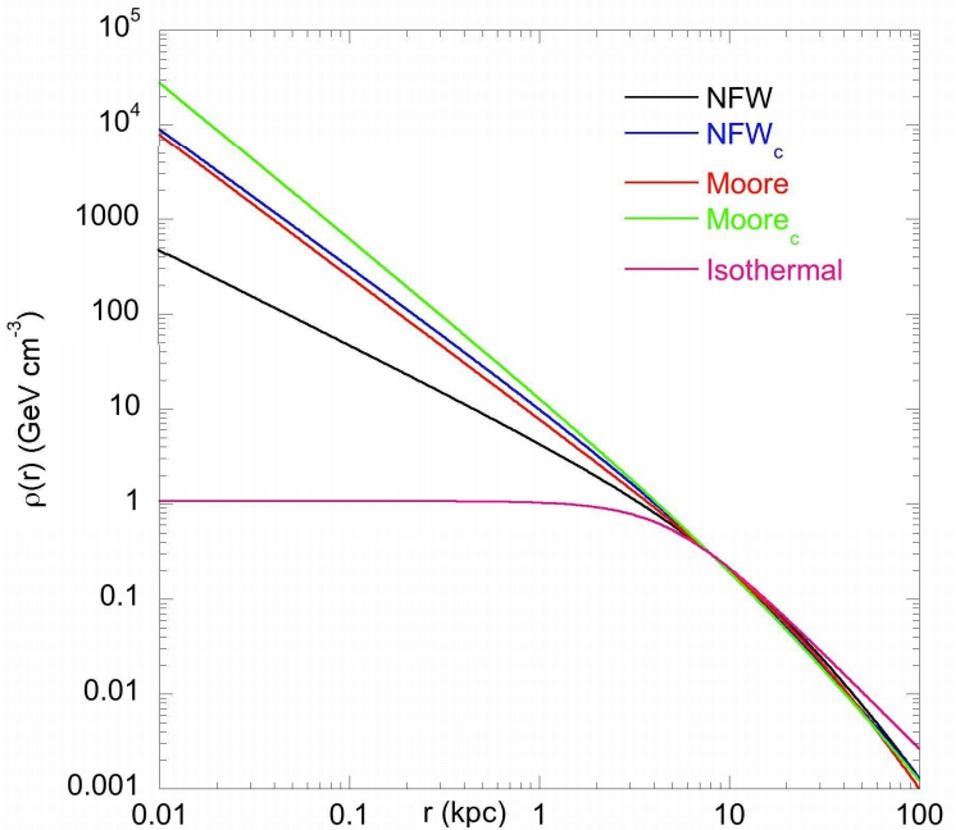
Moreover, the predictions of the universal profile are egregiously poor fits for low-mass galaxies in particular. It has led many to consider an alternative to dark matter — such as modifying the law of gravity — as those modifications can often lead to better predictions for the properties of individual galaxies as compared with dark matter’s predictions. Of course, those modifications do not work for larger-scale phenomena, and require something else, such as dark matter or a field that behaves indistinguishably from it, to explain those observations as well.
Although we’ve never directly detected dark matter and may never do so, dependent on its nature and properties, the fact is that no modified gravity theory can account for all of the observations without either including some type and amount of dark matter, or something else (like a field that clusters) that behaves indistinguishably from dark matter.
That’s why it’s so interesting to look at the systems — low-mass galaxies, and in particular, ultra-diffuse low-mass galaxies — that deviate the most from our standard “universal profile” dark matter predictions. If dark matter is anything other than truly cold and collisionless, we should be able to probe this from an observational perspective, and find out what the data points toward as far as how dark matter may not be cold and collisionless. In physics, we absolutely hate to invoke something novel unless we have no other alternative, and with these ultra-diffuse dwarf galaxies, there’s a lot to unpack.
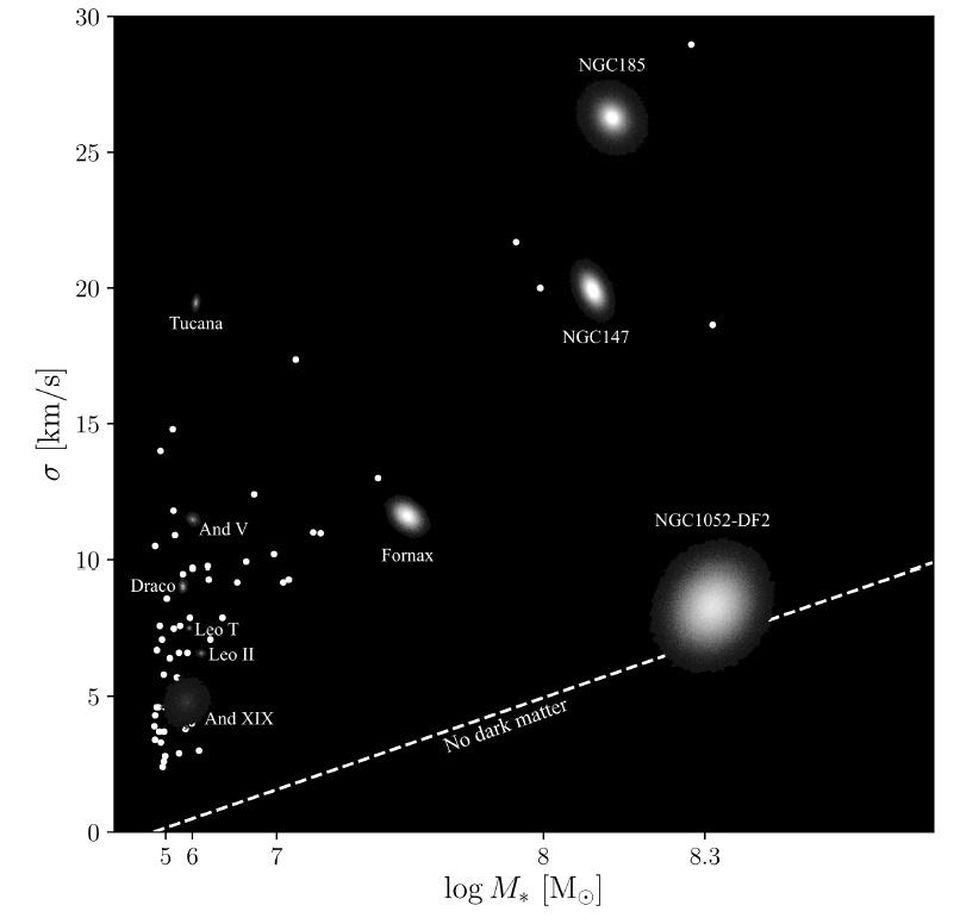
What we see is that, for galaxies above a certain mass, the dark matter to normal matter ratio, as inferred from the observations of internal motions of gas and stars within individual galaxies, remains at that same five-to-one ratio that is the hallmark of not only large-scale features in the Universe, but that is theorized to exist on the largest cosmic scales of all. However, as we go to smaller and smaller scales — i.e., as we start looking at lower-mass galaxies — we see that this trend no longer continues. Below masses of about 100 million solar masses (just ~0.01% of the mass of our galaxy, for example), we start to see deviations: where the lower the mass of the galaxy is, the greater the ratio of dark matter to normal matter.
For galaxies of tens of millions of solar masses, we see a ratio that’s more like ten-to-one or even a few dozen-to-one. For galaxies of even lower masses, that ratio can be more like a hundred-to-one. The most extreme examples include galaxies like Segue 1 and Segue 3, which have only around 1000 stars inside (with a total stellar mass of only a few hundred solar masses), but which have those stars speeding around inside at speeds that indicate a total mass for the galaxy of several hundred thousand solar masses. At the lowest masses, it seems like the density enhancements are most severe, but this probably isn’t due to dark matter being something other than collisionless; another explanation fits better.
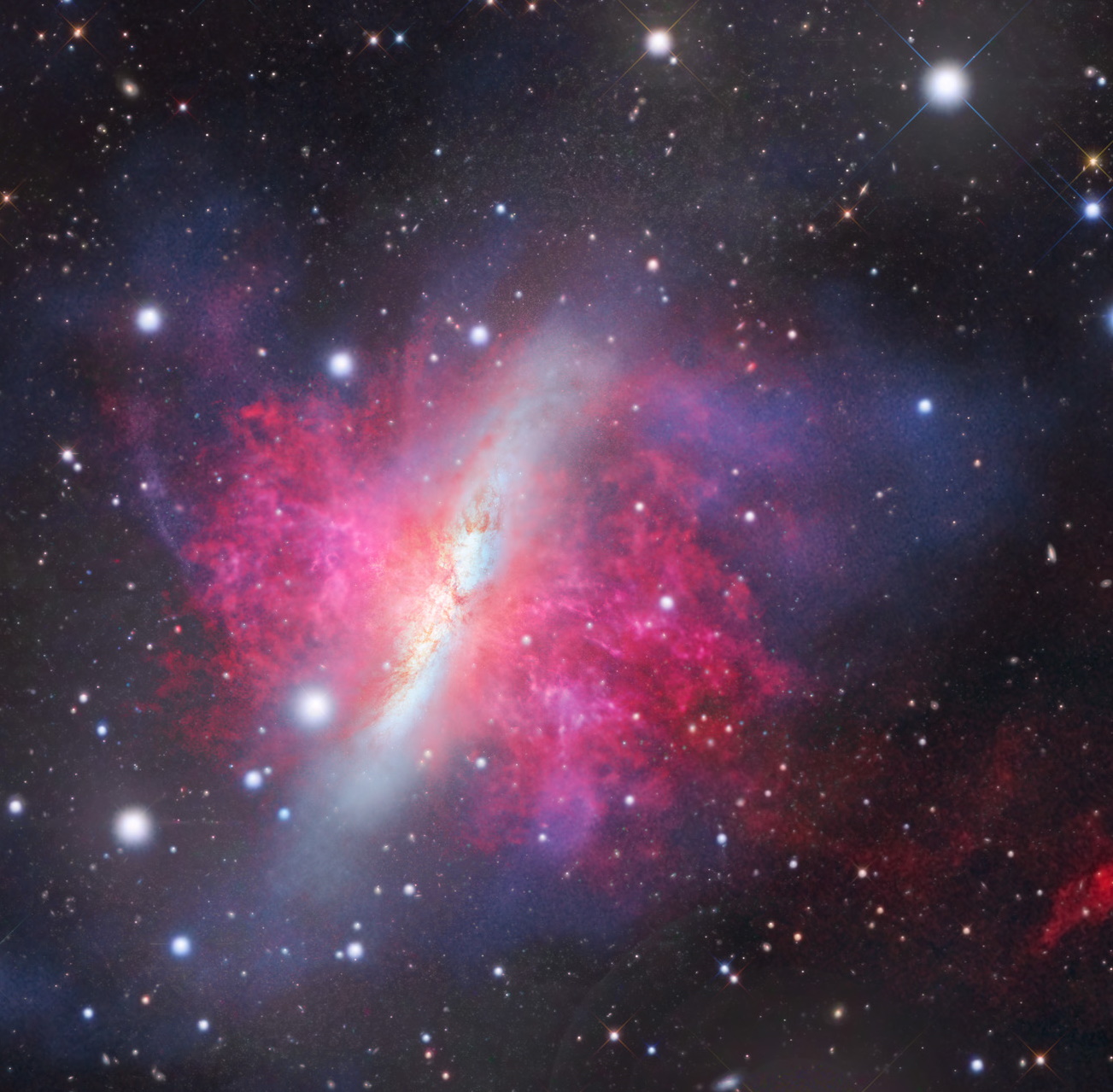
That’s because the act of star-formation itself is a violent process. When gas clouds collapse to trigger an episode of star-formation, they do so unevenly, fragmenting into many different clumps of material and igniting nuclear fusion in those protostellar cores at a variety of different times. In a big galaxy like the Milky Way, there’s a cosmic race at play between two competing factors:
- gravitation, which works to form and grow these clumps and to lead to the presence of new stars,
- and the radiation and winds from the newborn stars themselves, which pushes out on all of the gaseous matter and strips it away from newly forming stellar and protostellar systems.
Once the heaviest, most massive newborn stars begin dying in cataclysms like core-collapse supernovae, it spells the endgame for the gaseous matter, and in short order, stars stop forming entirely, as the source of that star-forming matter becomes depleted.
But in low-mass galaxies, the situation is far more dire: the matter that gets pushed out by winds and radiation can get “kicked” to great enough speeds that they can escape entirely from the low galactic masses that confine them. This can, if it’s efficient enough, eject all of the non-stellar matter, leading to a gas-free state for the galaxy: enhancing the dark matter-to-normal matter ratio by expelling normal matter. Then, once the shortest-lived, highest-mass stars die, only the longer-lived, lower mass stars remain amidst a galactic ocean of dark matter and (invisible) stellar remnants. In other words, the leading theory for why the dark matter-to-normal matter ratio is enhanced in low-mass galaxies provides no insights as to the nature of dark matter itself.

But this is not the only issue with “extreme” galaxies posing difficulties for dark matter. A new challenge comes in the form of ultra-diffuse galaxies: where the normal matter isn’t concentrated in the form of stars in the central region of a galaxy, but where the stars are distributed more evenly throughout it — including at great distances from the galactic center — despite a relatively low overall mass. When we look at this class of galaxies, the ultra-diffuse galaxies, we find that the “universal profile” fit for dark matter is worse than ever. Not only does it fail to show evidence for a “cusp” in the inferred dark matter density profile, but it instead points to a scenario where the dark matter’s central density is diffuse and approaches a constant value.
This is important, it should be noted, because that feature is incompatible with simulations of dark matter particles on its own. In a new paper authored by Jorge Sánchez Almeida, Ignacio Trujillo, and Angel R. Plastino, they show that six ultra-faint dwarf galaxies, which are also ultra-diffuse and which have only a few thousand solar masses worth of stars inside of them, all display features consistent with a flat central density profile, and that are inconsistent with a cuspy density profile, as predicted by dark matter simulations. They assert that this means that either dark matter is not collisionless or that the cold dark matter paradigm doesn’t work at all, stating:
“Thus, the evidence suggests that collisions among DM particles or other alternatives to CDM are likely shaping these galaxies.”
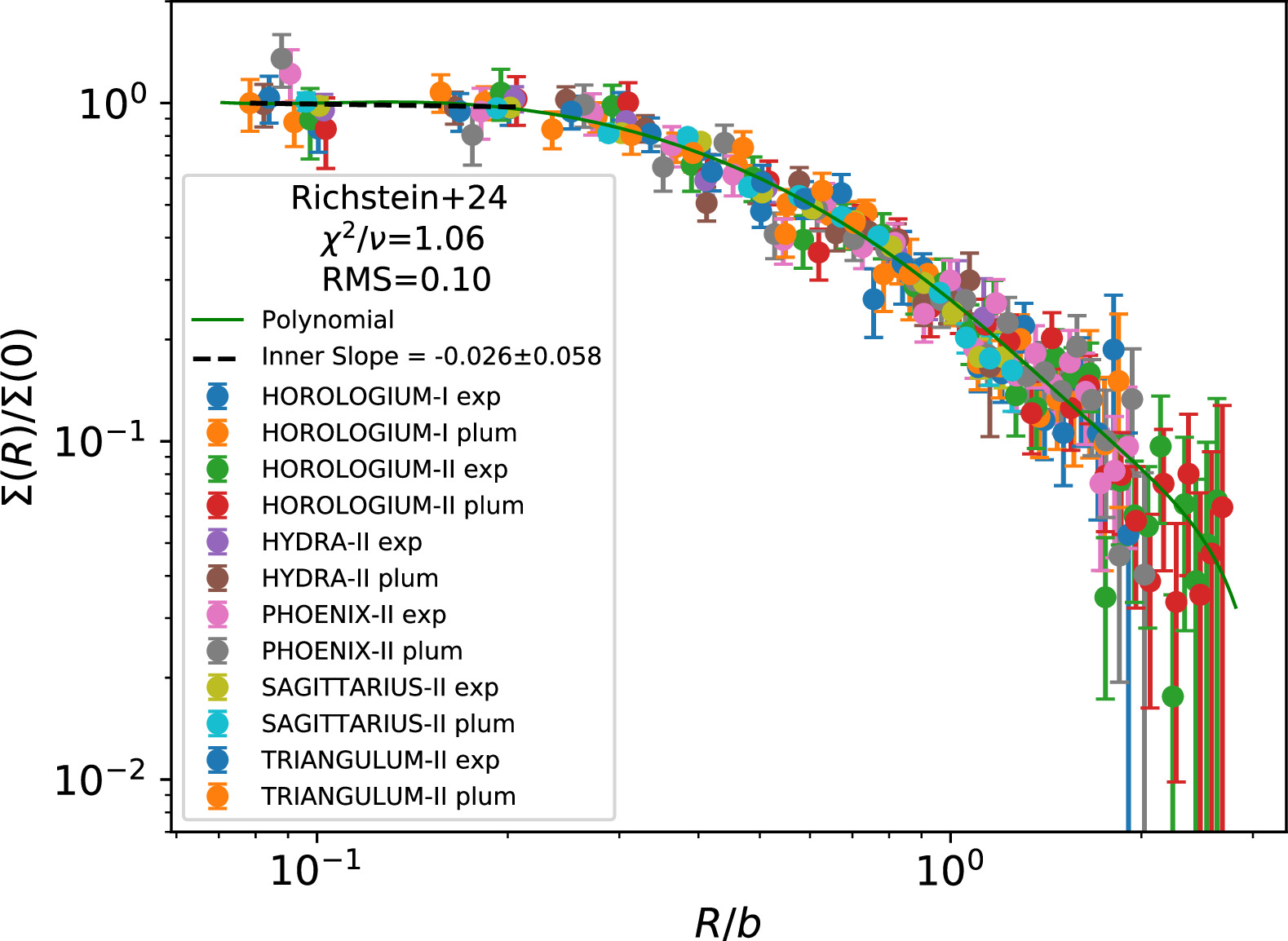
It’s vital to recognize that no one is doubting these observed facts. The stars within these galaxies — and indeed, within many other species of galaxies of low stellar masses — are tracing out the gravitational distribution of total matter: we can learn how the cumulative mass (dark matter and normal matter combined) inside is distributed as a function of distance from the galactic center, based solely on the motions of the stars we observe. However, the authors are quick to rush to a conclusion that may not be correct. According to Almeida:
“Using a novel technique based solely on the distribution of stars, we have been able to reject the collisionless cold dark matter model with high statistical significance… this work, therefore, provides a solid observational basis from which to explore more complex dark matter models.”
His collaboration, Trujillo, then adds:
“One of the most fascinating aspects of this study is that all the galaxies studied have an identical stellar distribution. It seems that the galaxies have forgotten their own history. This can only be well understood if dark matter has erased these galaxies’ past, indicating that its intimate nature is much more complex than we thought.”
It’s important to recognize this is one possible explanation: that dark matter isn’t interacting the way a cold, collisionless species of matter would be expected to interact. It’s reasonable to consider a scenario where dark matter does exhibit self-interactions, for example, and this could explain why the stars within these ultra-diffuse galaxies are tracing out something vastly different from what simulations predict.
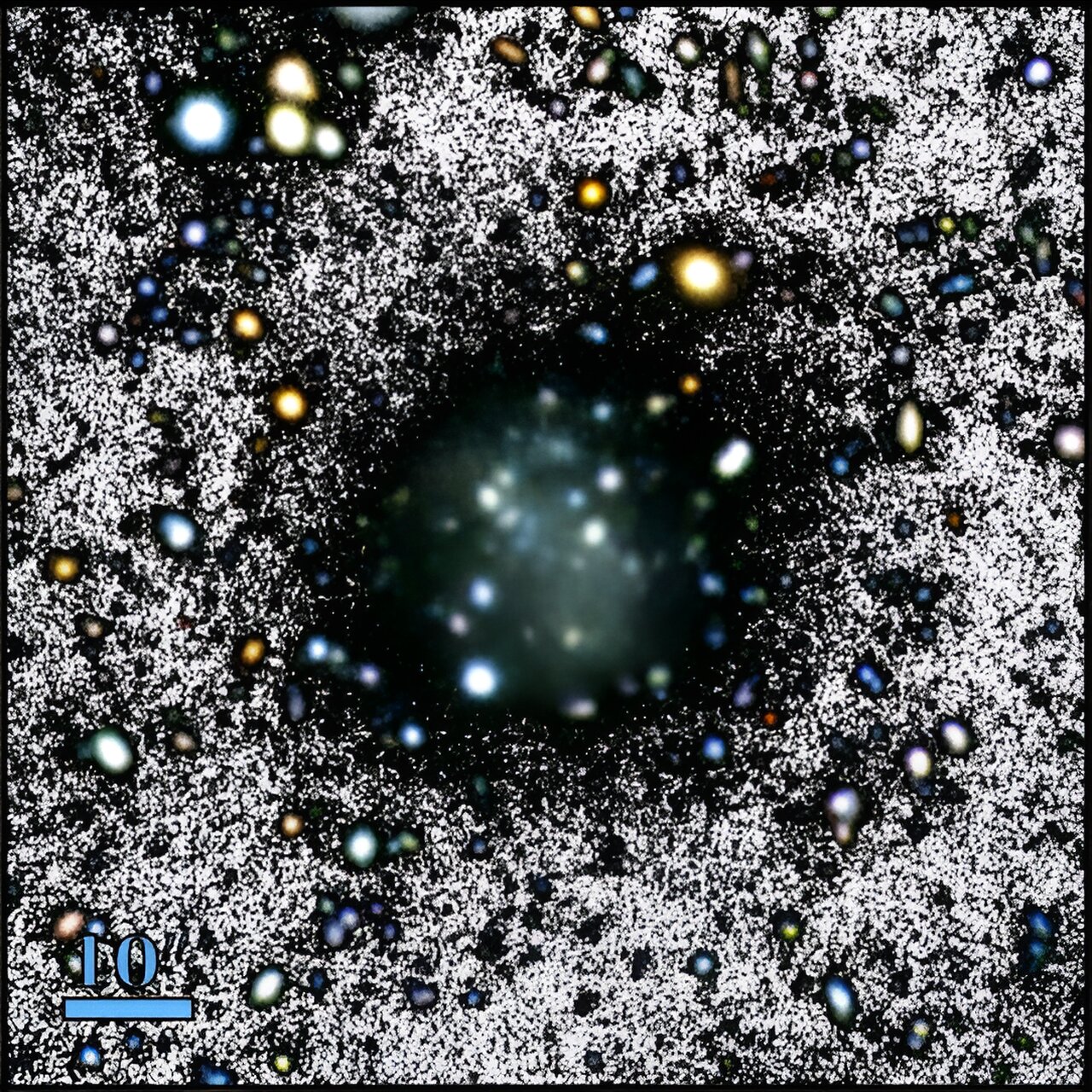
But there’s another option as well: one that might not require dark matter to be anything unexpected. These simulations that lead to the “universal profile” for dark matter halos traditionally neglect the effects of gas and stars; at just one-sixth (or less) of the total mass of these galaxies, the normal stuff is easy to overlook. However, gas and stars — i.e., the normal matter in these galaxies — can “heat up” even cold, collisionless dark matter, particularly at the centers of galaxies, from purely gravitational effects. As dark matter researcher Justin Read once related to me about this very topic,
“…radiation pressure, stellar winds and supernovae push the gas (via the usual electromagnetic interaction) and dark matter then responds to the altered central gravitational potential.”
This is expected to play an enormous role in faint, low-mass, and diffuse dwarf galaxies, even in cases like these where, as Trujillo correctly observes, the stars within these galaxies have “forgotten” their own history.
Ideally, we would like to simulate, end-to-end, how the normal matter and dark matter within a galaxy interact. We would like to have a comprehensive knowledge of the star-formation history within these galaxies, and the ability to model and simulate how the changing distribution of the normal matter over time, including from:
- gravitational collapse of normal matter,
- star-formation episodes,
- feedback from radiation and winds,
- and the eventual ejection of gas due to stellar cataclysms and other stellar processes,
affects the distribution of dark matter over our entire 13.8 billion year cosmic history. Alas, our simulations — still limited by computational power — cannot probe the full suite of necessary scales to incorporate all of these effects; we can only make model-dependent estimates.
It is possible, and perhaps (to some) suggested by the evidence, that dark matter may indeed be collisional or may interact in some fashion other than purely gravitationally. But with such a disconnect of so many steps between what we can theoretically predict versus what we can go out and directly observe, it’s far too early to reach a conclusive verdict on whether the standard paradigm of cold, collisionless dark matter is in any danger at all from these ultra-diffuse systems.