Forget About Electrons And Protons; The Unstable Muon Could Be The Future Of Particle Physics
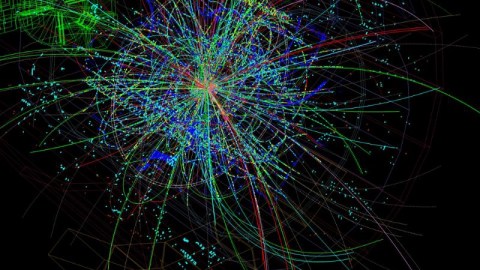
Electron-positron or proton-proton colliders are all the rage. But the unstable muon might be the key to unlocking the next frontier.
If you want to probe the frontiers of fundamental physics, you have to collide particles at very high energies: with enough energy that you can create the unstable particles and states that don’t exist in our everyday, low-energy Universe. So long as you obey the Universe’s conservation laws and have enough free energy at your disposal, you can create any massive particle (and/or its antiparticle) from that energy via Einstein’s E = mc².
Traditionally, there have been two strategies to do this.
- Collide electrons moving in one direction with positrons moving in the opposite direction, tuning your beams to whatever energy corresponds to the mass of particles you wish to produce.
- Collide protons in one direction with either other protons or anti-protons in the other, reaching higher energies but creating a much messier, less controllable signal to extract.
One Nobel Laureate, Carlo Rubbia, has called for physicists to build something entirely novel: a muon collider. It’s ambitious and presently impractical, but it just might be the future of particle physics.
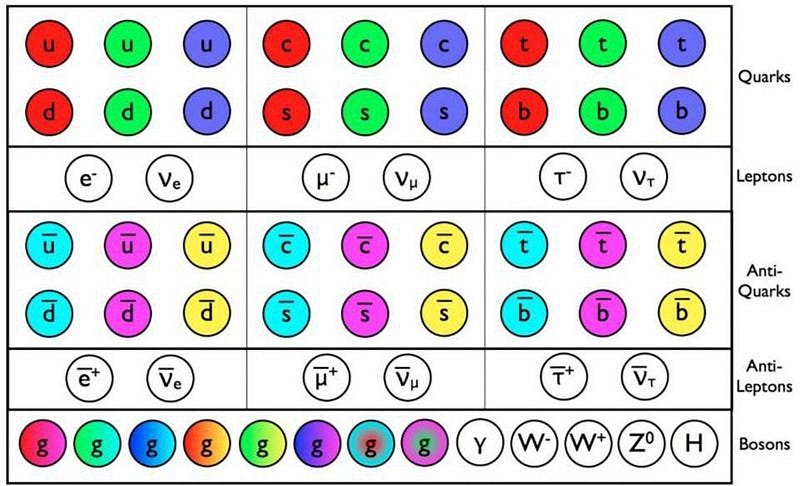
Above, you can see the particles and antiparticles of the Standard Model, which have now all been discovered. The Large Hadron Collider (LHC) at CERN discovered the Higgs boson, the long-sought-after last holdout, earlier this decade. While there’s still much science left to be done at the LHC — it’s only taken 2% of all the data it will acquire by the end of the 2030s — particle physicists are already looking ahead to the next generation of future colliders.
All of the plans put forth involve scaled-up version of existing technologies that have been used in past and/or current accelerators. We know how to accelerate electrons, positrons, and protons in a straight line. We know how to bend them into a circle, and maximize both the energy of the collisions and the number of particles colliding per second. Larger, more energetic versions of existing technologies are the simplest approach.
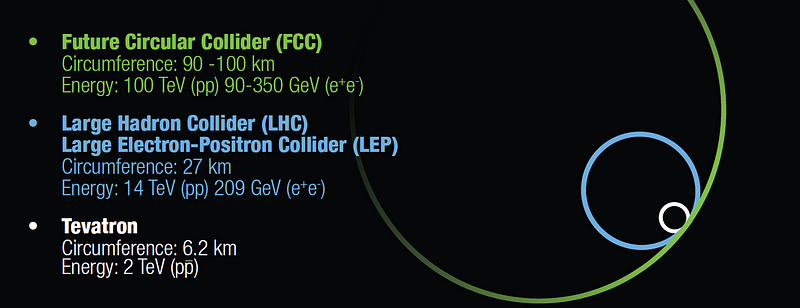
Of course, there are both benefits and drawbacks to each method we could use. You can build a linear collider, but the energy you can reach is going to be limited by how powerfully you can impart energy to these particles per-unit-distance as well as how long you build your accelerator. The drawback is that, without a continuous injection of circulating particles, linear colliders have lower collision rates and take longer amounts of time to collect the same amount of data.
The other main style of collider is the style currently used at CERN: circular colliders. Instead of only getting one continuous shot to accelerate your particles before giving them the opportunity to collide, you speed them up while bending them in a circle, adding more and more particles to each clockwise and counterclockwise beam with every revolution. You set up your detectors at designated collision points, and measure what comes out.
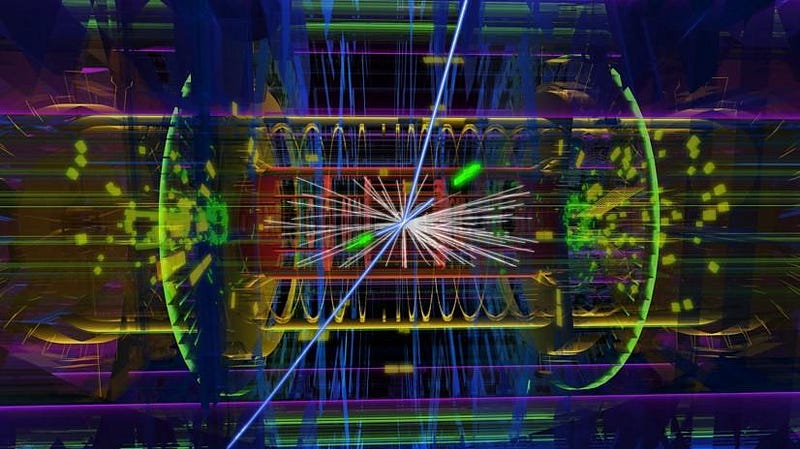
This is the preferred method, so long as your tunnel is long enough and your magnets are strong enough, for both electron/positron and proton/proton colliders. Compared to linear colliders, with a circular collider, you get
- greater numbers of particles inside the beam at any one time,
- second and third and thousandth chances for particles that missed one another on the prior pass through,
- and much greater collision rates overall, particularly for lower-energy heavy particles like the Z-boson.
In general, electron/positron colliders are better for precision studies of known particles, while proton/proton colliders are better for probing the energy frontier.
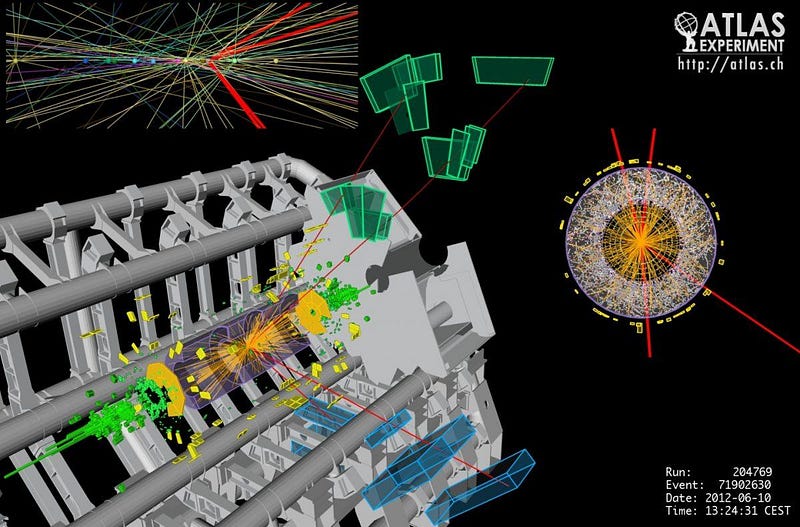
In fact, if you compare the LHC — which collides protons with protons — with the previous collider in the same tunnel (LEP, which collided electrons with positrons), you’d find something that surprises most people: the particles inside LEP went much, much faster than the ones inside the LHC!
Everything in this Universe is limited by the speed of light in a vacuum: 299,792,458 m/s. It’s impossible to accelerate any massive particle to that speed, much less past it. At the LHC, particles get accelerated up to extremely high energies of 7 TeV per particle. Considering that a proton’s rest energy is only 938 MeV (or 0.000938 TeV), it’s easy to see how it reaches a speed of 299,792,455 m/s.
But the electrons and positrons at LEP went even faster: 299,792,457.9964 m/s. Yet despite these enormous speeds, they only reached energies of ~110 GeV, or 1.6% the energies achieved at the LHC.
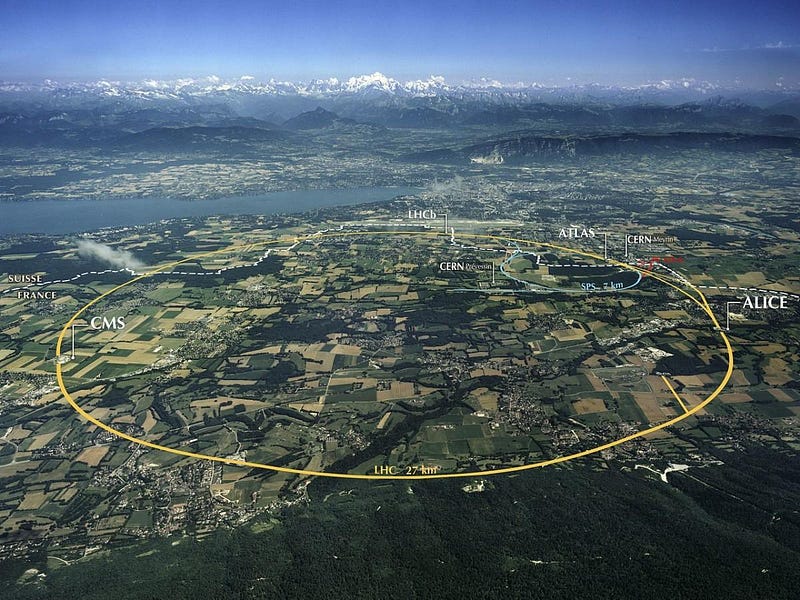
Let’s understand how colliding particles create new ones First, the energy available for creating new particles — the “E” in E = mc² — comes from the center-of-mass energy of the two colliding particles. In a proton-proton collision, it’s the internal structures that collide: quarks and gluons. The energy of each proton is divided up among many constituent particles, and these particles zip around inside the proton as well. When two of them collide, the energy available for creating new particles might still be large (up to 2 or 3 TeV), but isn’t the full-on 14 TeV.
But the electron-positron idea is a lot cleaner: they’re not composite particles, and they don’t have internal structure or energy divided among constituents. Accelerate an electron and positron to the same speed in opposite directions, and 100% of that energy goes into creating new particles. But it won’t be anywhere near 14 TeV.
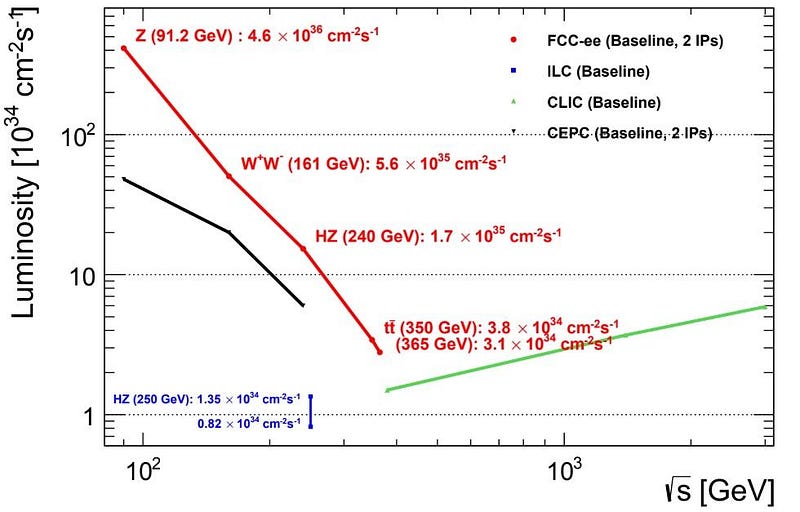
Even though electrons and positrons go much faster than protons do, the total amount of energy a particle possesses is determined by its speed and also its original mass. Even though the electrons and positrons are much closer to the speed of light, it takes nearly 2,000 of them to make up as much rest mass as a proton. They have a greater speed but a much lower rest mass, and hence, a lower energy overall.
There’s a good physics reasons why, even with the same radius ring and the same strong magnetic fields to bend them into a circle, electrons won’t reach the same energy as protons: synchrotron radiation. When you accelerate a charged particle with a magnetic field, it gives off radiation, which means it carries energy away.
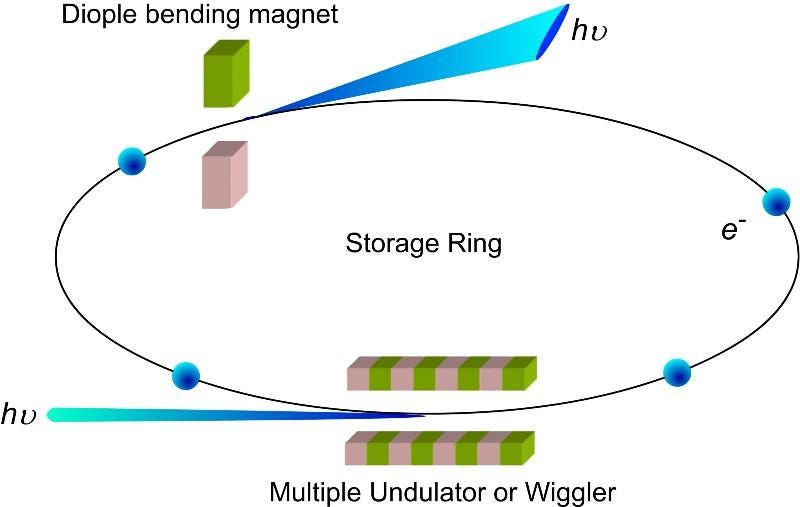
The amount of energy radiated away is dependent on the field strength (squared), the energy of the particle (squared), but also on the inherent charge-to-mass ratio of the particle (to the fourth power). Since electrons and positrons have the same charge as the proton, but just 1/1836th of a proton’s mass, that synchrotron radiation is the limiting factor for electron-positron systems in a circular collider. You’d need a circular collider 100 km around just to be able to create a pair of top-antitop quarks in a next-generation particle accelerator using electrons and positrons.
This is where the big idea of using muons comes in. Muons (and anti-muons) are the cousins of electrons (and positrons), being:
- fundamental (and not composite) particles,
- being 206 times as massive as an electron (with a much smaller charge-to-mass ratio and much less synchrotron radiation),
- and also, unlike electrons or positrons, being fundamentally unstable.
That last difference is the present dealbreaker: muons have a mean lifetime of just 2.2 microseconds before decaying away.
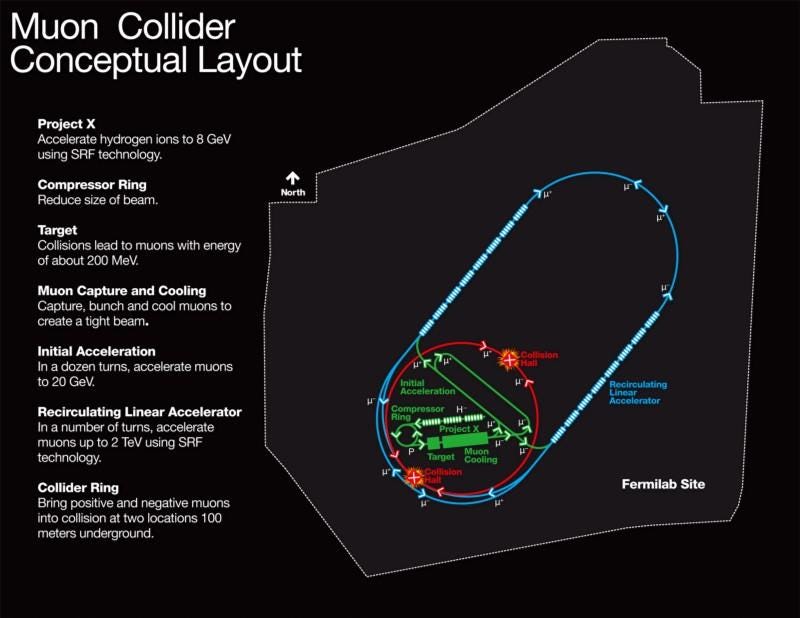
In the future, however, we might be able to work around that anyway. You see, Einstein’s special relativity tells us that as particles move closer and closer to the speed of light, time dilates for that particle in the observer’s reference frame. In other words, if we make this muon move fast enough, we can dramatically increase the time it lives before decaying; this is the same physics behind why cosmic ray muons pass through us all the time!
If we could accelerate a muon up to the same 6.5 TeV in energy that LHC protons achieved during their prior data-taking run, that muon would live for 135,000 microseconds instead of 2.2 microseconds: enough time to circle the LHC some 1,500 times before decaying away. If you could collide a muon/anti-muon pair at those speeds, you’d have 100% of that energy — all 13 TeV of it — available for particle creation.
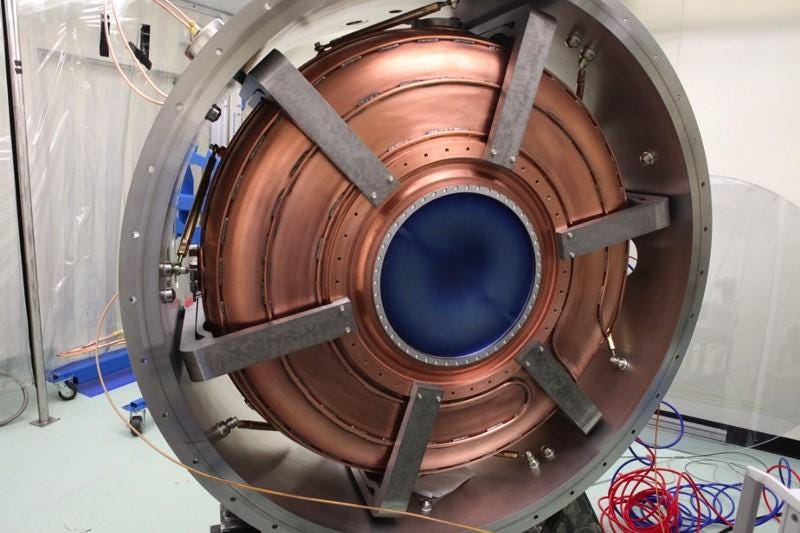
Humanity can always choose to build a bigger ring or invest in producing stronger-field magnets; those are easy ways to go to higher energies in particle physics. But there’s no cure for synchrotron radiation with electrons and positrons; you’d have to use heavier particles instead. There’s no cure for energy being distributed among multiple constituent particles inside a proton; you’d have to use fundamental particles instead.
The muon is the one particle that could solve both of these issues. The only drawback is that they’re unstable, and difficult to keep alive for a long time. However, they’re easy to make: smash a proton beam into a piece of acrylic and you’ll produce pions, which will decay into both muons and anti-muons. Accelerate those muons to high energy and collimate them into beams, and you can put them in a circular collider.
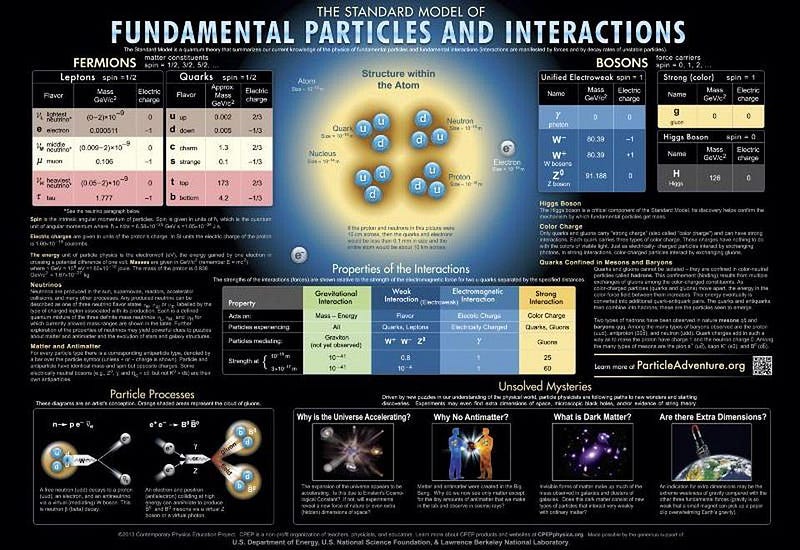
The MICE collaboration — which stands for Muon Ionization Cooling Experiment — continues to push this technology to new heights, and may make a muon collider a real possibility for the future. The goal is to reveal whatever secrets nature might have waiting in store for us, and these are secrets we cannot predict. As Carlo Rubbia himself said,
these fundamental choices are coming from nature, not from individuals. Theorists can do what they like, but nature is the one deciding in the end.
Ethan Siegel is the author of Beyond the Galaxy and Treknology. You can pre-order his third book, currently in development: the Encyclopaedia Cosmologica.