Can the new-and-improved Large Hadron Collider save particle physics?
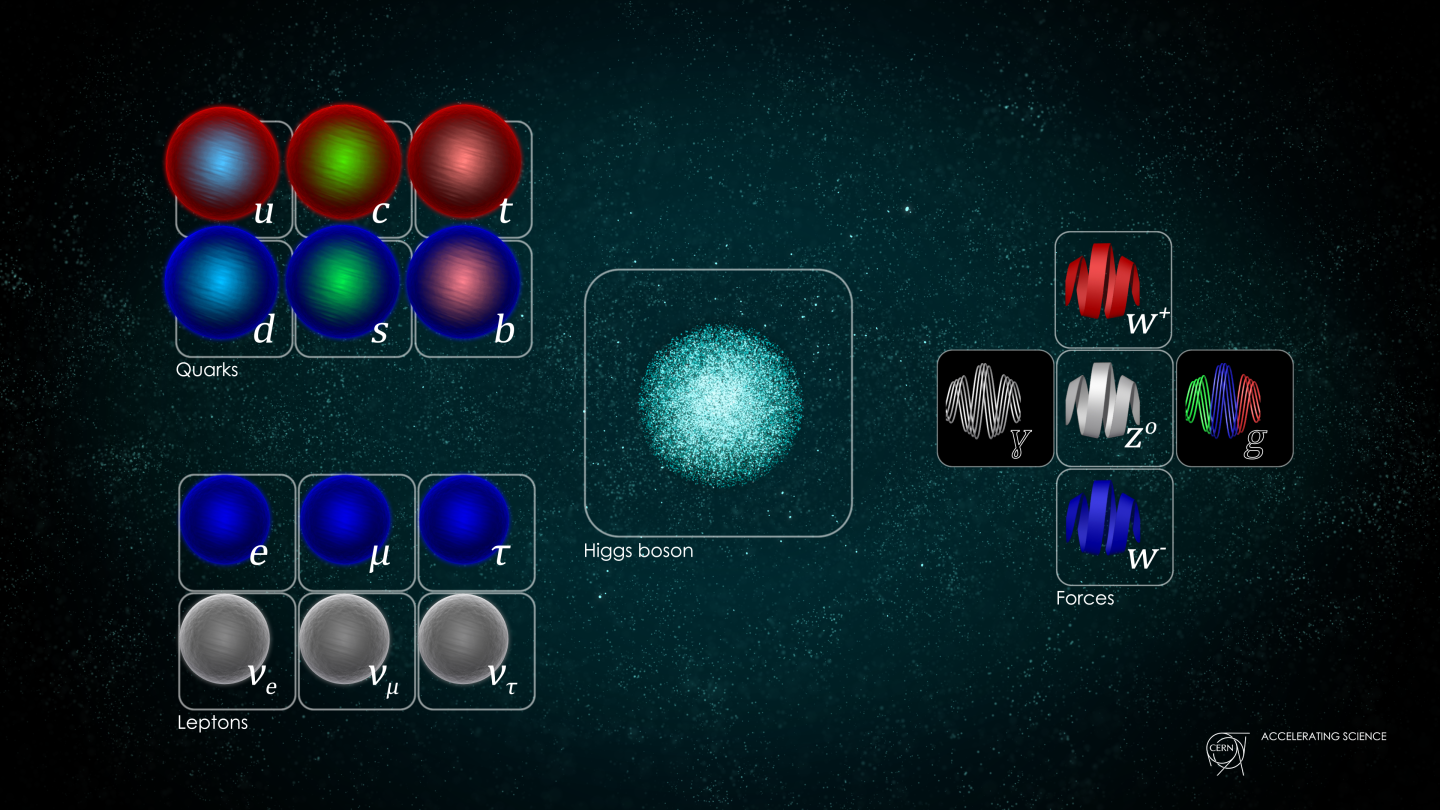
- The Standard Model of particle physics is the most successful physics theory in history, and the only “cracks” in it are contentious, relatively new, and unconfirmed.
- The Large Hadron Collider, after finding the Higgs Boson but nothing else fundamentally new, just restarted after being shut down for over three years.
- With more proton-proton collisions than ever before, even though the energy of those collisions hasn’t increased, there’s every reason to hope it’ll save experimental particle physics.
Everyone in the physics community agrees: particle physics is in trouble. Yet, depending on whom you ask, you’re likely to hear that the root of the trouble originates in a few very different places.
- Particle physics is in trouble because there are now so many cracks in the Standard Model that it might well be time to throw out our most cherished assumptions and start over.
- Particle physics is in trouble because theorists have led us astray for generations, and we’ve continued to barrel down the same path.
- Particle physics is in trouble because the Large Hadron Collider has only found the expected Higgs Boson while failing to find anything else new, and still particle physicists want a newer and bigger accelerator.
But the truth of the matter isn’t any of these; these are all convenient but incomplete narratives that deliberately leave out the most important aspects of what’s going on. The truth is that there’s much more to learn beyond the already-explored frontiers, and the value of that knowledge is not knowable until we go out and find it. There’s a tremendous case for not only starting to build the next particle accelerator now, but also to pay very close attention to what the LHC discovers during the next crucial decade. Here’s the story you aren’t getting elsewhere.
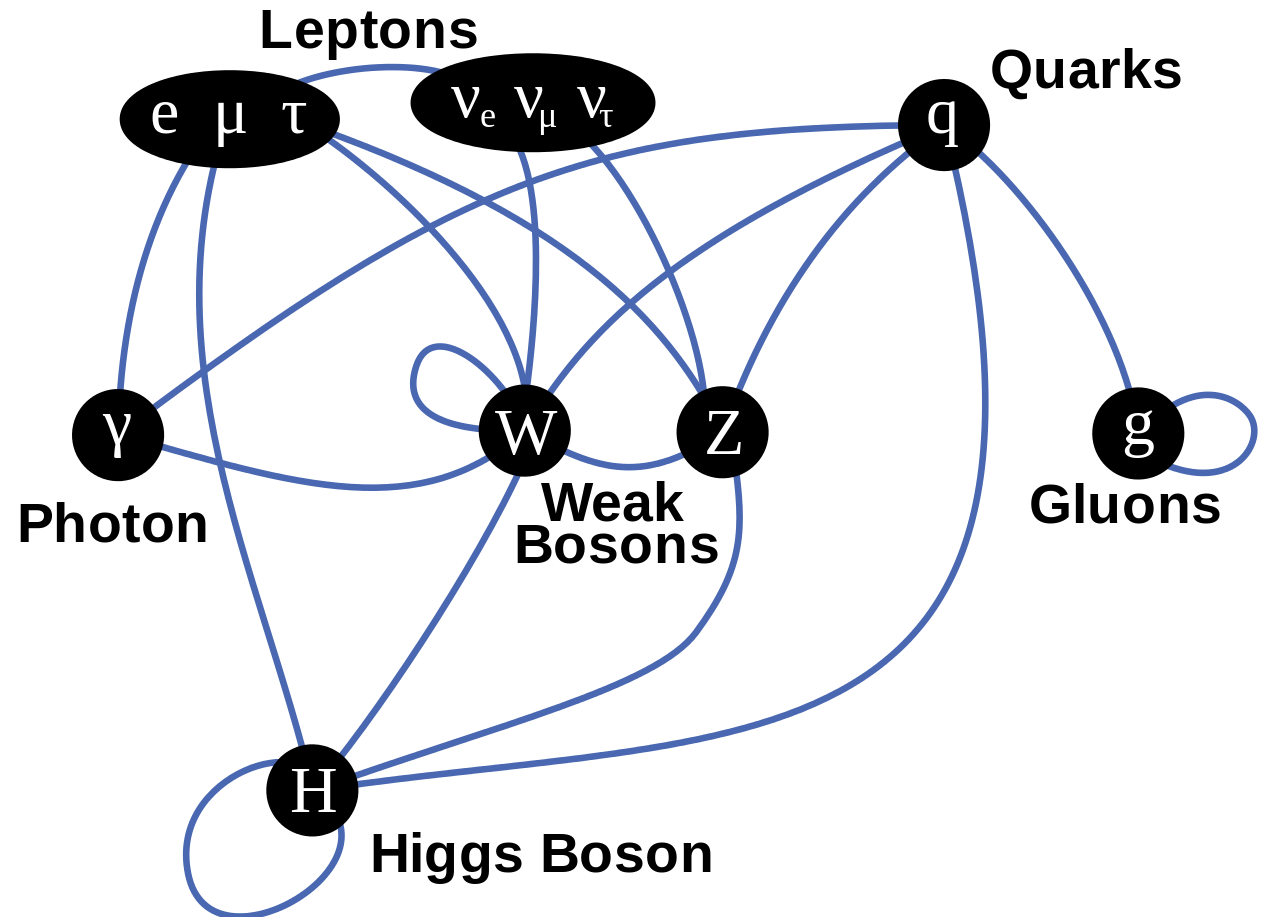
Particle physics has two sides to it: a theoretical side and an experimental side. On the theoretical side, there’s the Standard Model, which is arguably the most successful physical theory of all-time, and then there are ideas for what lies out there beyond the Standard Model. The Standard Model is a predictive framework for understanding the particles in our Universe, what their properties are, how they interact and decay, and what we expect the results of any specific laboratory experiment to yield. There are also extensions to the Standard Model — possible ways to go beyond it — that have been explored at length over the past 50+ years.
The Standard Model tells us what particles there are and how they couple to one another, and allows us to determine not only what interactions are possible versus forbidden, but what the relative probability of each potential outcome is. There are many ways to put the Standard Model to the test, but arguably the most effective is to collide two particles moving at the highest equal-and-opposite velocities as possible, over and over, as many times as you can, and detect what comes out. By measuring the debris of this collision precisely enough, you can reconstruct what happened at every step of the way.
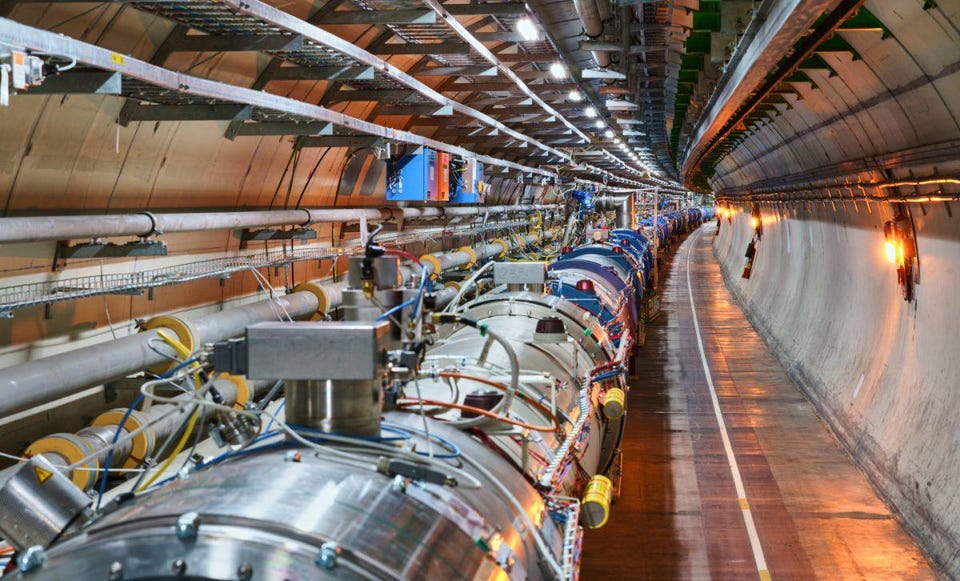
The Large Hadron Collider (LHC) at CERN is the most powerful particle accelerator in Earth’s history, capable of reaching energies of approximately 7 TeV per particle. That equates to accelerating protons to 299,792,455 m/s apiece, just 3 m/s shy of the maximum achievable speed in the Universe: the speed of light. For years, since it first turned on in 2008, the LHC has been colliding as many protons with other protons by:
- bunching them together,
- circulating them in both the clockwise and counterclockwise directions in its large ring,
- timing their motions so that the bunches intersect at particular “collision points,”
- where large detectors are awaiting those collisions,
- and they measure, at a series of distances, the energy, momentum, charge, spin, and other properties of the particles that arise in the aftermath of that collision.
By measuring what comes out of those collisions and comparing those experimental results with the theoretical predictions that arise from both Standard Model-only scenarios as well as beyond-the-Standard Model scenarios, you can determine how well the Standard Model, by itself, fits what you’ve observed. Any departures from it, so long as they’re robust enough, are something that will make the entire community sit up and take notice.
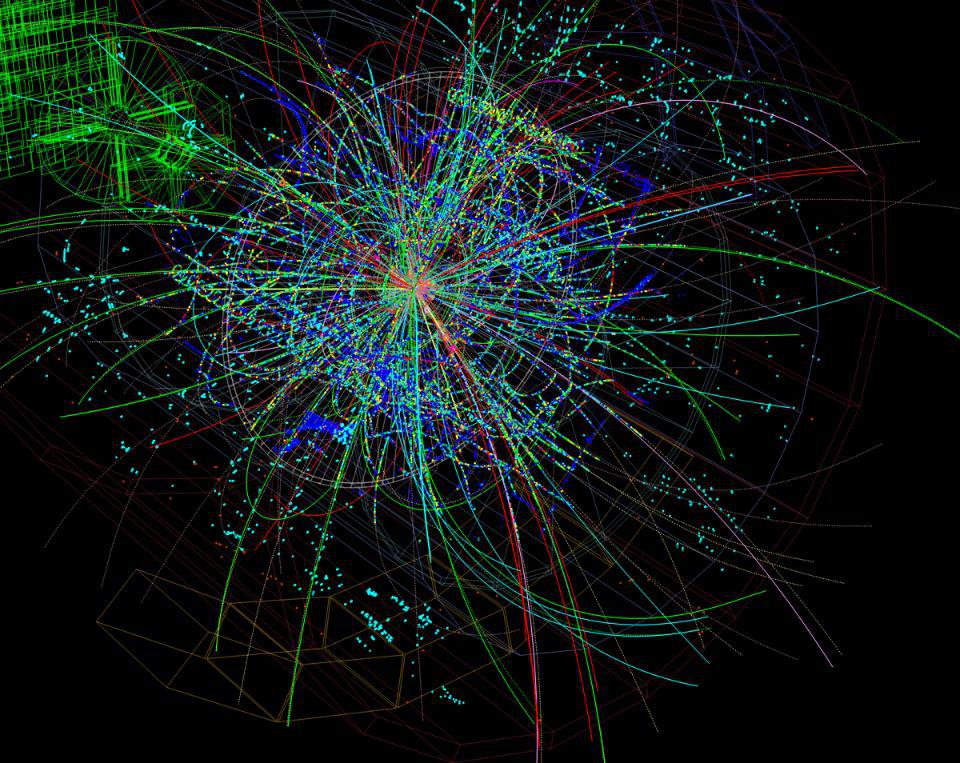
Prior to the LHC, we had discovered some remarkable cracks in the façade of the Standard Model: things that came as surprises to us.
- We discovered that neutrinos and antineutrinos weren’t massless and didn’t remain the same species of particle, but rather had a non-zero rest mass and would oscillate from one flavor (electron, mu, tau) to the others, particularly when they interacted with matter.
- We discovered that dark matter exists, and were able to rule out all of the Standard Model particles (as the Standard Model predicts them) as viable candidates for it.
- We discovered the accelerated expansion of the Universe, which we attribute to a new form of energy (dark energy), which again cannot be explained by anything within the Standard Model.
- We knew that there was no CP-violation in the strong interactions; only in the weak interactions, despite the fact that the Standard Model allows for both. If a spinning particle decays, the antiparticle counterpart that spins in the opposite direction is observed to behave differently under the weak interactions, but not the strong interactions, and nobody knows why.
- And finally, we live in a Universe made predominantly of matter and not antimatter, but the laws of physics do not have enough of an asymmetry between them to create the matter-rich Universe we inhabit today.
In many ways, even before the Large Hadron Collider turned on for the first time, we knew that there must be more to the Universe than the Standard Model gives us.
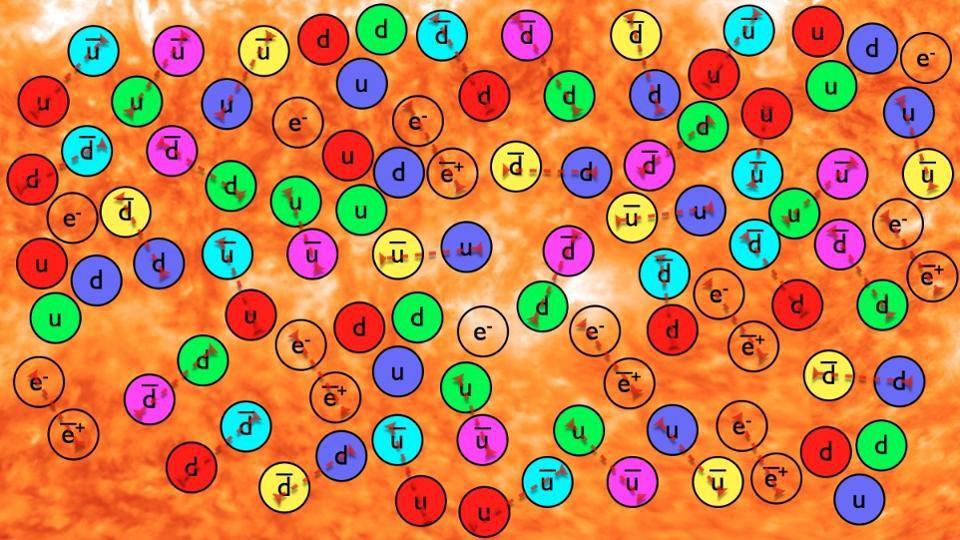
But at particle colliders, where we take matter (and sometimes antimatter), smash it together at high energies, and observe the particle debris that comes out, there have been no observations or measurements that have stood the test of time that run counter to the Standard Model’s predictions. When the LHC began colliding particles, it was the last good hope for many theoretical ideas that had been bandied about as plausible explanations for what could be out there beyond the Standard Model for many decades.
Ideas like supersymmetry, extra dimensions, technicolor, supergravity, grand unification, and string theory-inspired extensions to the Standard Model have all been heavily in fashion for some 40-odd years in most cases. And yet, there were only ever-tightening constraints on those scenarios. There were a few false alarms that came out of the LHC’s early results — tentative evidence for a “diboson bump” which could have been a new particle at 2-3 TeV that evaporated; evidence for a “diphoton bump” which could have meant a new particle at about 700 GeV that also fell away with more data; evidence for faster-than-light neutrinos that turned out to be an equipment error — but nothing held up. With more and better data, all of these results were shown to be mere statistical flukes.

It’s now been a full decade since the Higgs boson was discovered, and it was determined to have only the properties predicted by the Standard Model. Thus far, there’s no new accelerator evidence, directly or indirectly, for any fundamental particles that aren’t accounted for by the Standard Model, and for any interactions or decays that aren’t predicted by the Standard Model.
Given that this is the case, you can find many, many pessimistic takes on the field of particle physics as a whole, and find many — physicists and non-physicists alike — who view large colliders as an expensive folly whose time ought to come to an end.
These takes are all not only disingenuous, presenting a heavily slanted and overall inaccurate version of the actual truth, but they miss the fundamental point of not just particle physics, but physics in general, as a scientific discipline. Physics is an experimental science, and the way you advance an experimental science is to subject the Universe to inquiries that you’ve never subjected it to before, and listen very closely and carefully to what it tells you. That’s how you learn more about the fundamental nature of reality.

This is true in every physical field.
- If you want to study the low-temperature Universe, you always strive for that next significant digit in your quest for absolute zero. From a few kelvin to millkelvin to microkelvin to nanokelvin and now down into the picokelvin range, each step colder reveals more properties of matter under extreme conditions than ever before.
- If you want to study the astrophysical Universe, you push the frontiers of what you can see in terms of depth (by building larger observatories), in terms of wavelength (by probing every range from gamma-rays down to radio waves), in terms of clarity (by going to space), and in terms of what you measure (by measuring not just light, but also cosmic particles and gravitational waves).
- And if you want to study the world of particles, you always strive to measure their properties and behavior in new realms (i.e., at higher energies) and with greater precision (i.e., with greater numbers of events) as compared to everything prior.
In other words, the two frontiers we need to be pushing, if we want to understand the Universe of particles better than we understand them today, are to probe the Universe at higher energies and/or at greater precisions — with more collisions — than ever before.
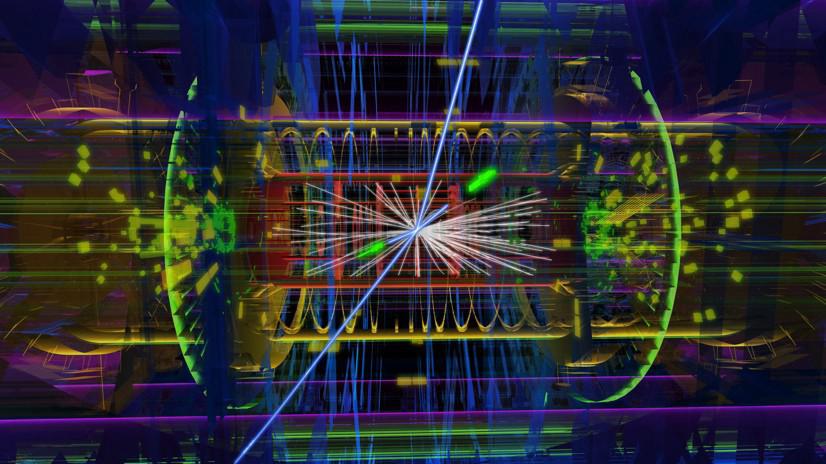
It’s possible to go to higher energies, but the only ways to achieve them are to either increase the physical size of the accelerator (which, realistically, requires building a new accelerator) or to increase the strength of your bending magnets. It’s historically been considered “worth it” to build a new accelerator when you can increase your energy by a factor of 7-to-10 or more, which is the energy leap that was made from Fermilab’s Tevatron to CERN’s LHC, and which is the planned leap from the current LHC to the proposed Future Circular Collider (FCC), the leading prospect for humanity’s next particle collider. It’s important to plan for that, but also to recognize that it will take more than a decade to construct it.
In the meantime, however, the LHC just completed a major part of an enormous upgrade to what’s being called the HL-LHC, or high-luminosity LHC. In astrophysics, luminosity refers to how bright an astrophysical source is; in particle physics, it’s how “luminous,” or rich-in-particles, the beam is. By creating more “bunches of particles” that collide more frequently and by increasing the number of particles that collide when two “bunches” cross each other, the current incarnation of the LHC has already broken the all-time luminosity record for particle colliders.
In fact, over the next 10-to-15 years, the LHC will take somewhere between 40 and 50 times as much data as it’s cumulatively taken so far, since its first turn-on in 2008. In other words, the LHC data we’ve collected and analyzed so far represents only about 2% of the total data that the LHC will wind up accumulating. The LHC measures the total amount of collected data in what we call “inverse femtobarns,” and had collected 150 inverse femtobarns by the close of operations in 2018. The HL-LHC will collect over 250 inverse femtobarns each year when it’s complete.
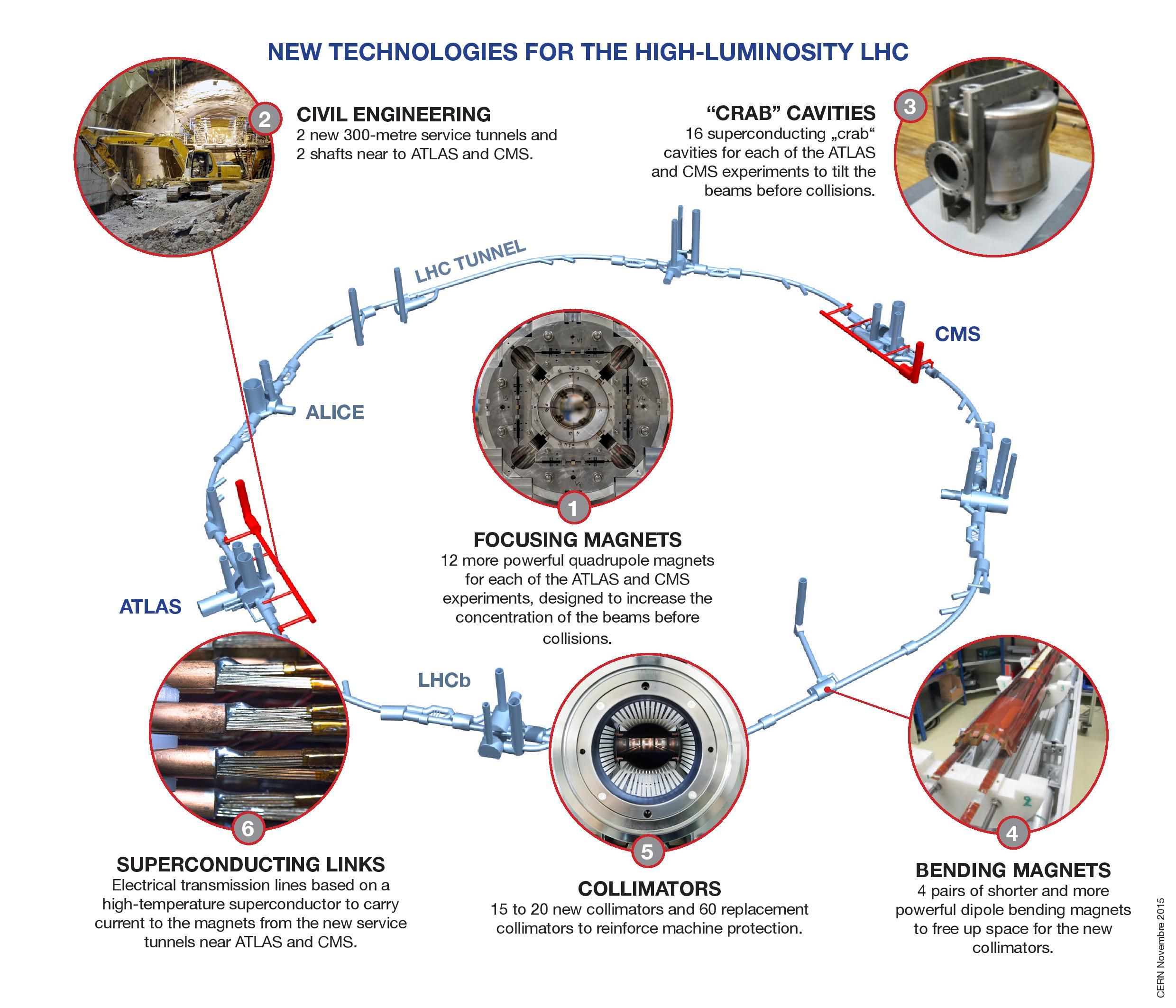
With that data, the LHC will be able to test the Standard Model in an unprecedented series of ways.
- They’ll be able to create greater numbers of Higgs bosons than ever before, testing whether it decays according to the Standard Model’s predictions to better than ~1-part-in-100 precision.
- They’ll be able to test lepton flavor universality, currently one of the three still-standing Standard Model anomalies (along with the W-boson’s mass and the muon’s anomalous magnetic moment), to unprecedented precision. If it’s a real problem with the Standard Model, this will undoubtedly push it over the “gold standard” 5-sigma threshold.
- They’ll be able to test whether the CDF collaboration’s measurement for the W-boson’s mass matches their value, with much more (albeit less clean) data than Fermilab was ever able to collect.
- They’ll be able to test top-quark and bottom-quark physics to better precision than ever, either confirming the Standard Model’s predictions or running counter to it.
And, perhaps most excitingly, they’ll look for clues of new physics in places where we might not expect it. With nearly 98% of the total data that the LHC will eventually take left to go, giving up on the possibility that it will find something worth writing home about is both unnecessarily defeatist and also wildly premature.
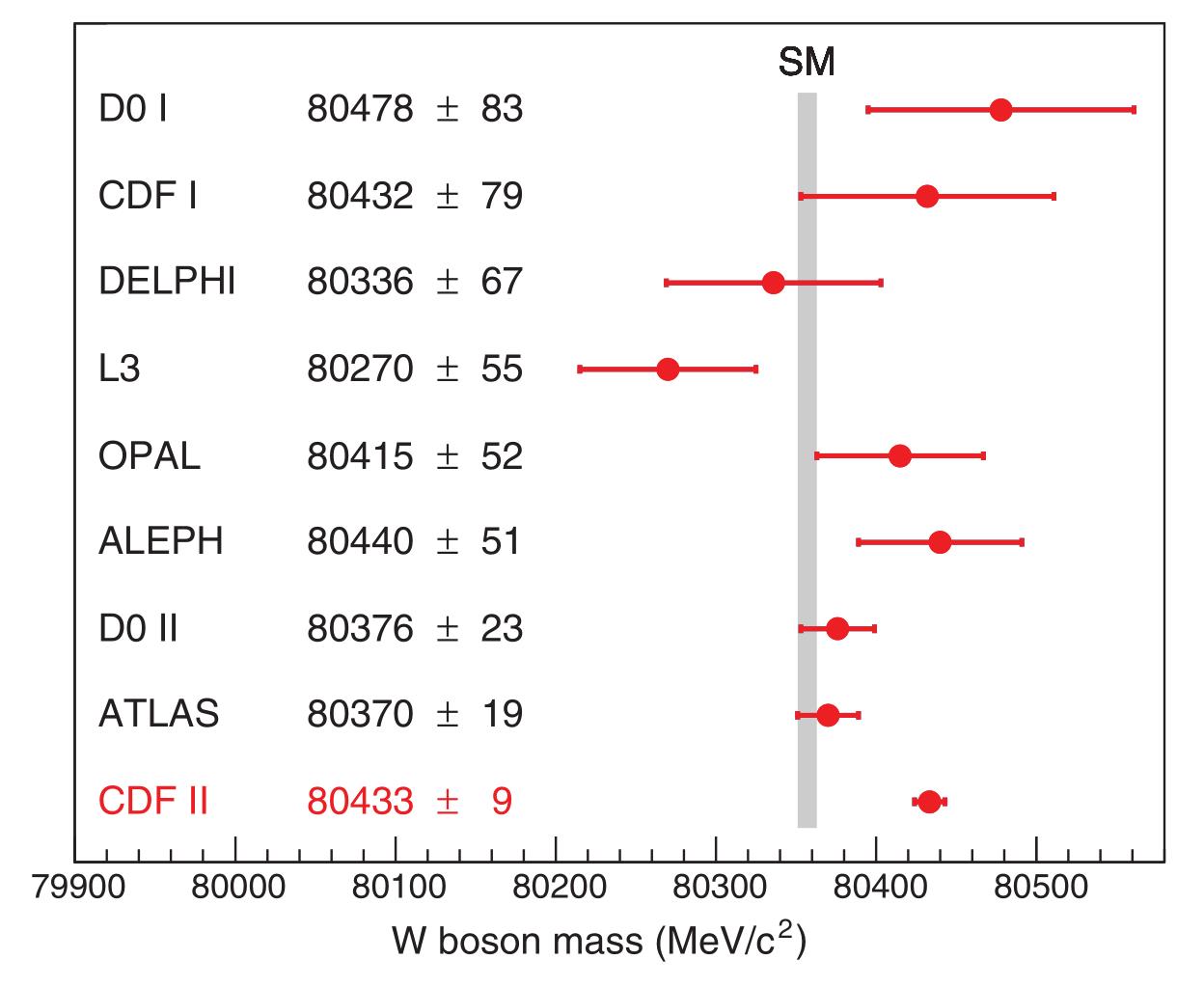
Sure, the expectations of theorists for what might lie beyond the Standard Model have turned out to be abysmal, as all of the scenarios preferred by the community are sorely lacking in any substantial evidence. But the failure of a detective to imagine what clues ought to be there is no excuse for giving up on the case.
Yes, it’s arguable that theorists have been doing nothing more than stabbing in the dark, and appear to have missed thus far with each and every strike.
Yes, the LHC hasn’t found a new fundamental particle beyond the Standard Model yet, and it’s plausible that it may not, even once 100% of the data has been taken.
And yes, there are a number of cracks in the Standard Model, and modern particle physics cannot account for a wide variety of observations concerning our Universe.
But the successes of particle physics cannot be ignored, and that’s a remarkable triumph of the Standard Model where all of its alternatives fail. In the quest for the next big breakthrough concerning the nature of reality, there’s no reason to expect that the fruit we seek will be on the lowest-hanging branches. But if we fail to climb the tree of knowledge and see what we discover all the way along our journey to the top, we’ll have only ourselves to fault. The game is not yet over, but we can be certain that the surefire losing move is not to play at all.