No, Physicists Still Don’t Know Why Matter (And Not Antimatter) Dominates Our Universe
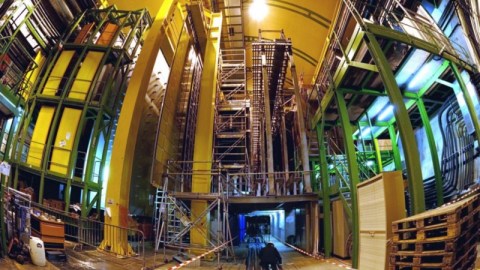
There is a fundamental difference between matter and antimatter. But not enough of one to explain our Universe.
Our Universe is a vast and enormous place, and if there’s one thing we can be certain of, space definitely isn’t empty. Everywhere we look, we find evidence for the same cosmic story: the Universe had a hot, dense past, was filled with nearly-equal amounts of matter everywhere, and grew up to form stars, galaxies, and a vast cosmic web as time progressed.
Although it’s a beautiful picture, it’s an incomplete one. While we know how the Universe creates atoms, stars, galaxies, planets and more, we don’t yet know why the Universe is full of matter. In physics, matter and antimatter are only ever produced or destroyed in equal amounts, so it’s a puzzle that our Universe is all matter and no antimatter. While a new result from the LHC at CERN is making big waves, it doesn’t solve this problem at all.
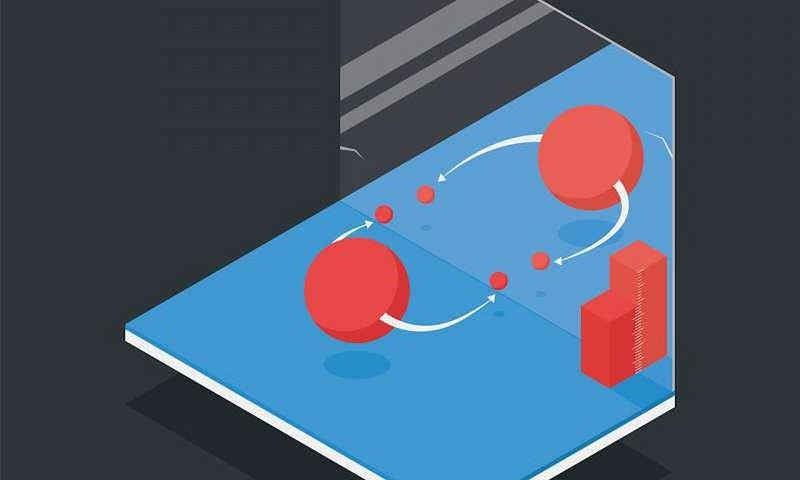
You might not realize this if all you read are the dubious headlines proclaiming, “Physicists Reveal Why Matter Dominates Universe.” After all, the puzzle of why our Universe is made of matter and not antimatter is one of the biggest unsolved problems in physics today. If we were to solve this puzzle, it would represent one of the greatest advances of all-time in our understanding of the Universe, and would surely win a Nobel Prize.
These latest results are interesting, as they do reveal a way that the Universe isn’t completely symmetric between matter and antimatter, which is an important component to the story. But, as you’ll see when we take a detailed look at the full picture, it doesn’t explain why matter dominates the Universe. Moreover, it doesn’t come close to answering the key question that keeps people working on this up at night: how did we make more matter than antimatter?
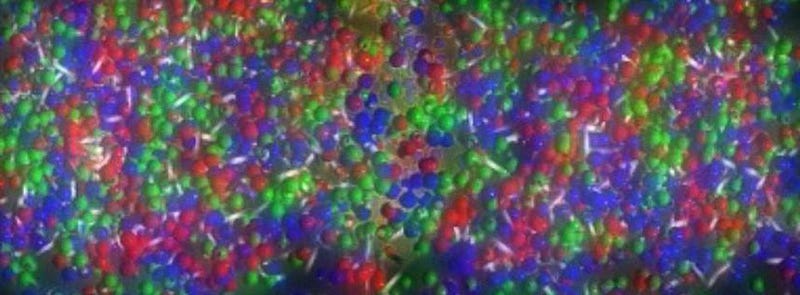
The first part to the puzzle is recognizing that this really is an existential problem. The Universe really is made of matter and not antimatter, and this is not a problem that’s going to go away. It isn’t the case that some parts of the distant Universe are made of antimatter and the Universe is really matter-antimatter symmetric; it isn’t plausible that the matter we see is due to a random, pro-matter (and anti-antimatter) fluctuation in the early Universe; it isn’t a problem that disappears if we hypothesize an equal-and-opposite antimatter Universe as a counterpart to our own.
Whenever and wherever antimatter and matter meet in the Universe, there’s a fantastic outburst of energy due to particle-antiparticle annihilation, and we don’t see that anywhere on large scales.
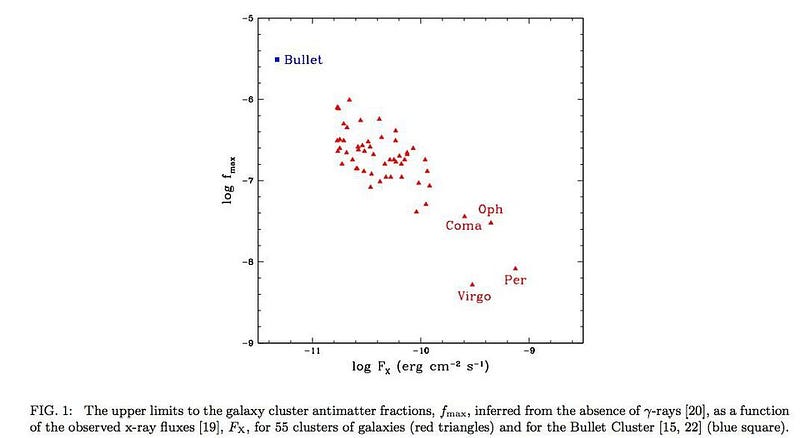
Moreover, the amount of matter we see is some 1010 times greater than any random fluctuation could cause. There’s too much matter all throughout our Universe, in too consistent a fashion, to simply be accounted for by any of these explanations.
Instead, we are compelled to look for a physical cause. That means we need to consider what physical scenarios could potentially generate a matter-antimatter asymmetry in our Universe that’s consistent with the total amount of matter we now know to be present. The endeavor to find out how this happened in our distant past — to understand the origin of the matter-antimatter asymmetry — is known as the problem of baryogenesis. We know that it must have happened a long, long time ago. The key challenge is to uncover how it unfolded.
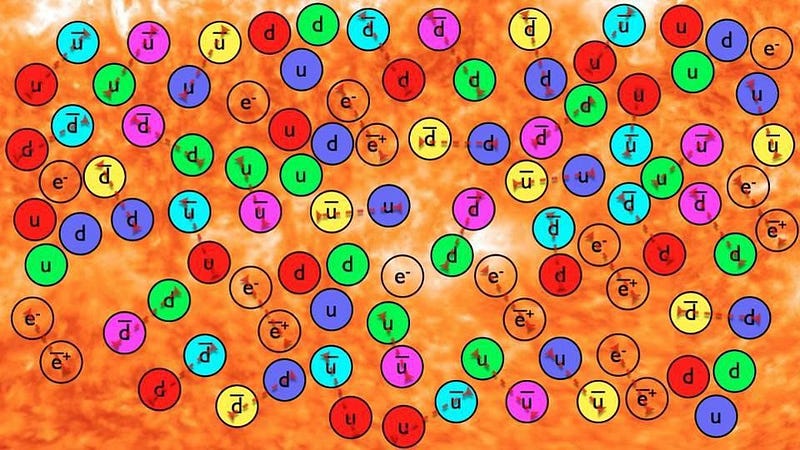
According to the hot Big Bang, the Universe as we know it today was born 13.8 billion years ago, and was filled with energy in the form of photons, particles, and antiparticles. The Universe was hot, dense, and expanding extremely rapidly under those early conditions, which caused the Universe to cool. By the time less than a single second had passed, practically all of the antimatter had annihilated away, leaving approximately 1 proton and 1 electron for every 1 billion photons.
The Universe was thought to be born matter-antimatter symmetric, as the laws of physics dictate. But something must have happened during that first fraction of a second to preferentially create matter and/or destroy antimatter, leaving an overall imbalance. By the time we get to today, only the matter survives.
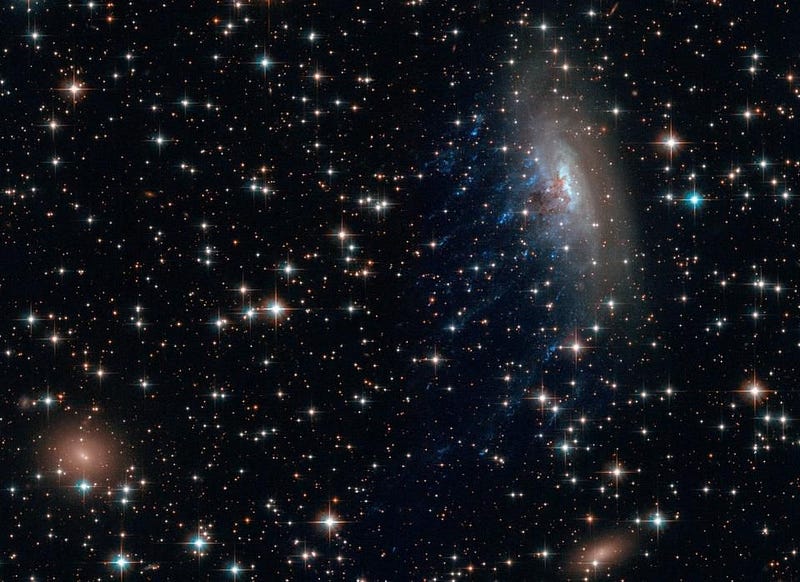
If our Universe somehow created a matter/antimatter asymmetry during these early stages, we should be able to figure out how it happened by looking to high-energy physics. Highly energetic interactions correspond to the high-temperature conditions present in the early Universe. Since the laws of physics remain unchanged over time, all we need to do is recreate those conditions and look for a possible cause of today’s asymmetry.
We’ve known how to create more matter than antimatter in theory since the late 1960s, when physicist Andrei Sakharov identified the three conditions necessary for baryogenesis. They are as follows:
- The Universe must be an out-of-equilibrium system.
- It must exhibit C– and CP-violation.
- There must be baryon-number-violating interactions.
That’s it.
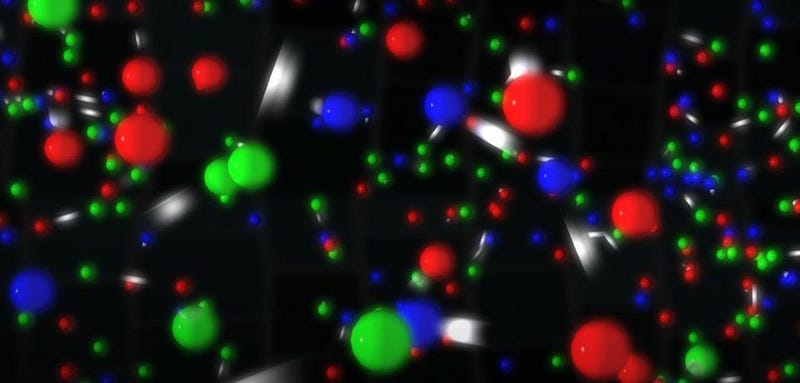
The first one is easy; if you live in a hot Universe that’s expanding and cooling, then by definition it’s an out-of-equilibrium system. Equilibrium only occurs if your system — like a large room, for example — has had enough time for all the different components in different locations to interact with each other, exchange information (like temperature), and come to a state where energy isn’t being transferred from one place to another.
It’s very easy to show that objects we can view many billions of light-years away on one side of the Universe have not yet, even now, had time to exchange information with equidistant objects in the opposite direction. The expanding Universe is perhaps the ultimate out-of-equilibrium system, and gives us reasons to hope that we can solve baryogenesis after all.
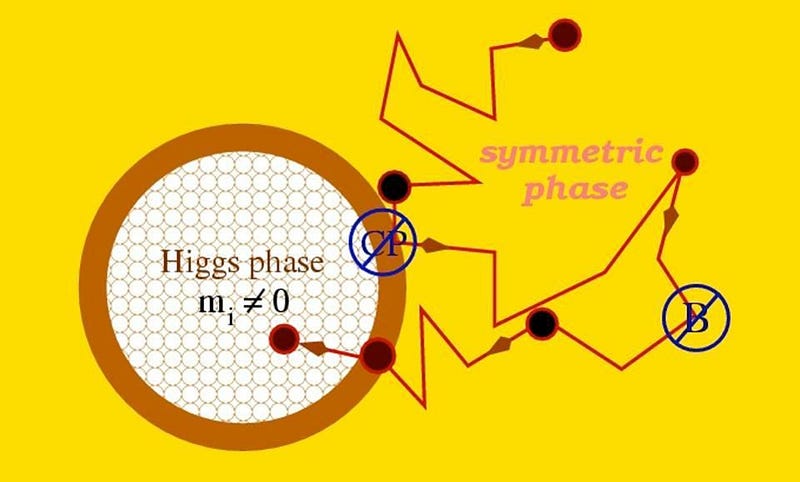
The second condition is more challenging. There are three fundamental symmetries in particle physics:
- Charge conjugation, or C-symmetry, which is what you get if you swap out particles for their antiparticles.
- Parity, or P-symmetry, which is what you’ll see if you reflect particles in a mirror.
- Time reversal, or T-symmetry, which is what you’d obtain if you ran the clock backwards instead of forwards.
You are allowed to violate any one or two of these in the Standard Model (e.g., C, P, or CP), although all three combined (CPT) must be conserved. In practice, only the weak interactions violate any of them; they violate Cand P in very large amounts, but violate CP together (and also T, separately) by only a little bit. In every interaction we’ve ever observed, CPT is always conserved.
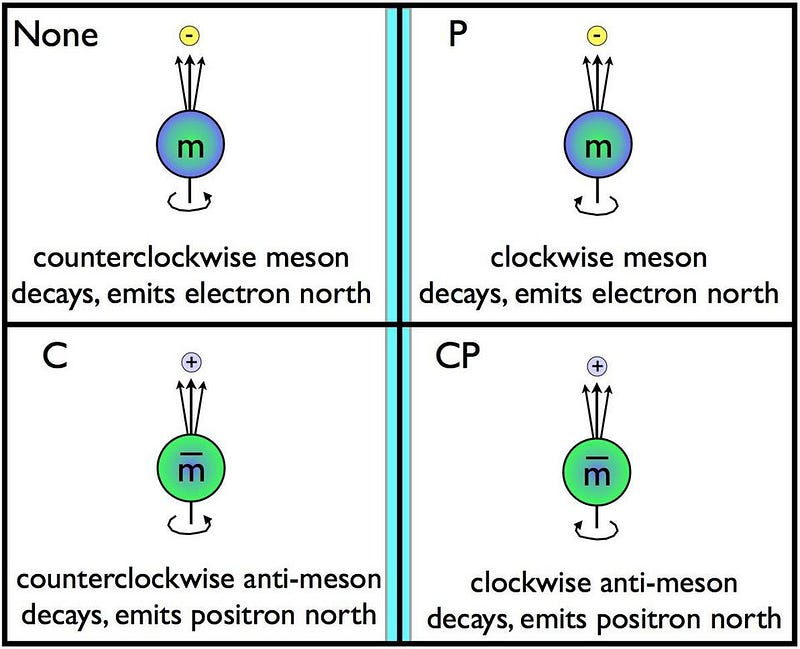
CP-violation was first observed in the neutral Kaon system: where particles known as mesons that were combinations of quark-antiquark pairs (specifically, made of down-antistrange and/or strange-antidown quarks) displayed certain differences in their particle properties. Since then, we’ve discovered CP-violation in composite particles that involve either strange, charm, or bottom quarks, or their antiquark counterparts.
The CP-violation observed recently was for particles that contain either up-anticharm quarks or charm-antiup quarks: the D0 and anti-D0particles. According to researcher Sheldon Stone:
“There have been many attempts to measure matter-antimatter asymmetry, but, until now, no one has succeeded. It’s a milestone in antimatter research.”
But don’t take that quote at face value. This is the first time the asymmetry has been measured, sure, for particles with charm quarks. It was already well-measured for strange and bottom-containing particles.
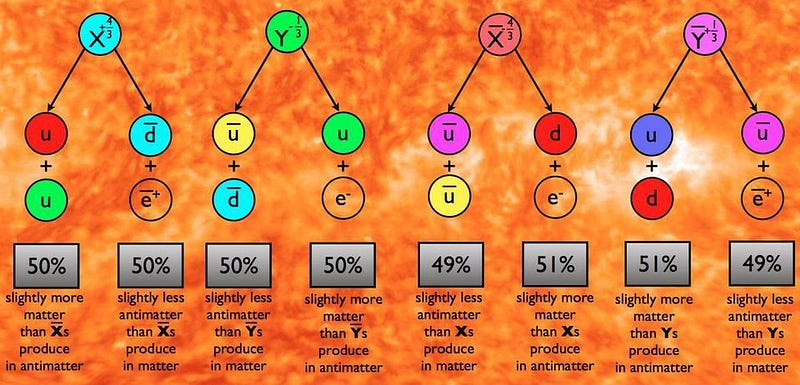
The big problem is not getting C- and CP-violation. The big problem is that, in the Standard Model, there aren’t enough baryon-number-violating interactions — the third of the three Sakharov conditions — for the amount of C– and CP-violation that we have. The amount of CP-violation we’ve found in these charmed mesons, the D0 and anti-D0, does remarkably little to help that.
We’re not short by a few percent or a factor of 2 or 10 or 100, either. We can produce a matter-antimatter asymmetry, but it’s too small by a factor of many millions, at least. We’d need to discover some sort of new physics at the electroweak scale, either in terms of extra C– and CP-violation or additional baryon-number-violating interactions, to explain the Universe we know we have today.
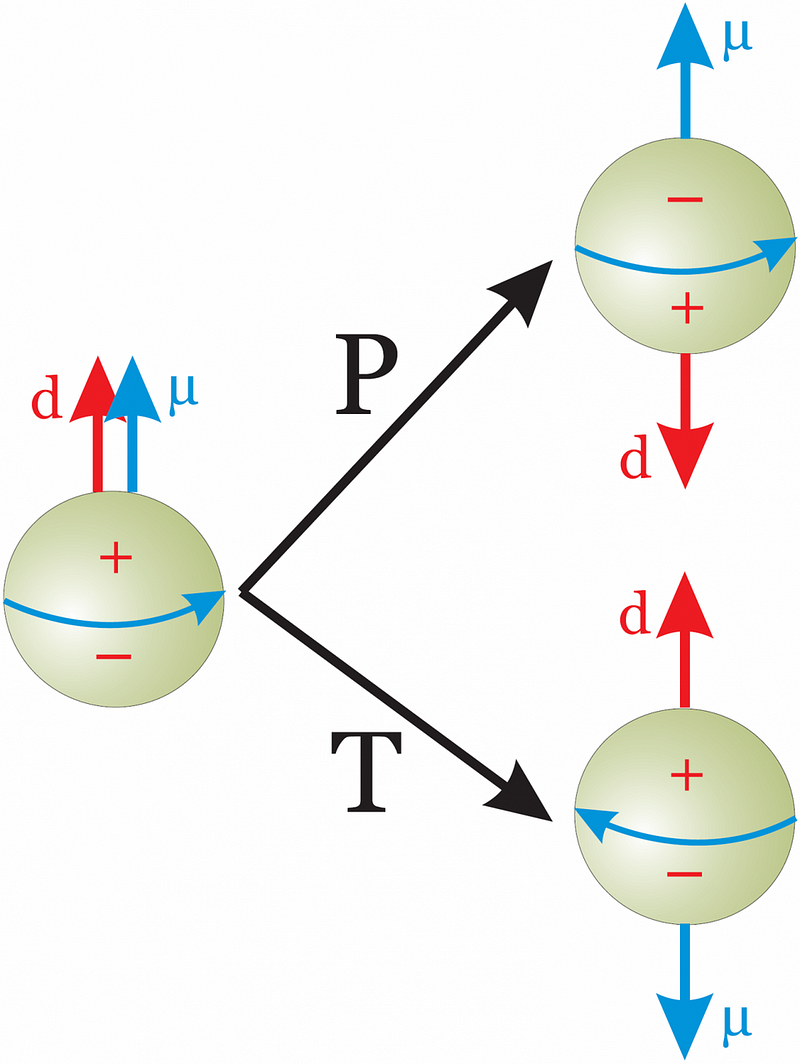
It’s a remarkable advance to have detected CP-violation in particles containing charm quarks and antiquarks, demonstrating once again that there are real, subtle differences between matter and antimatter. Specifically, if you compare the particle and antiparticle versions, you’ll find that although the total lifetimes are the same and they have identically corresponding decay pathways, the branching ratios of the decays differ.
If the version with the charm quark has a percentage decaying into A and another percentage decaying into B, the version with the charm antiquark will decay into anti-A and anti-B, but in slightly different percentages. The ~0.1% difference is similar to what has been seen in systems with strange and bottom quarks, and it’s a tremendous experimental achievement by scientists working at the LHCb experiment.
But why does the Universe possess the amount of matter we see, rather than less, or even none at all? We’re still no closer to that answer.
Ethan Siegel is the author of Beyond the Galaxy and Treknology. You can pre-order his third book, currently in development: the Encyclopaedia Cosmologica.