The simplest explanation for ultra-high-energy cosmic rays
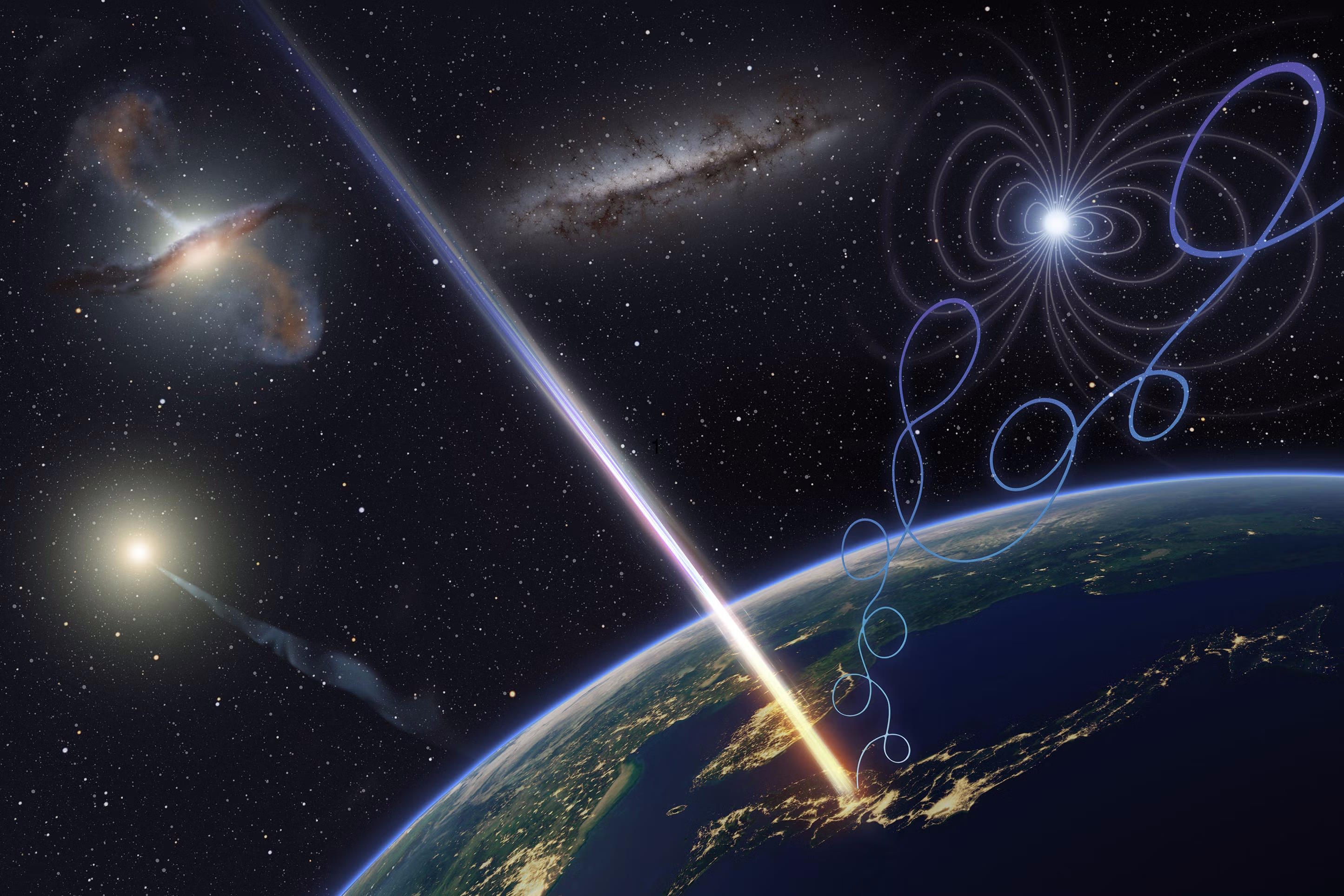
- From all across the Universe, from our Sun, from neutron stars, black holes, and sources well beyond our galaxy, high-energy cosmic particles continuously strike the Earth.
- In 1991, a particle of unprecedented energy was detected: known as the “Oh My God” particle for how spectacular it was. No similar particle was ever seen again… until May 21, 2021.
- If it wasn’t a mistake or a bug, what could the explanation for these ultra-high-energy cosmic rays be? While some easily speculate about new physics, the simplest explanation is truly iron-ic.
Earth, whether we like it or not, serves as a cosmic particle detector on a continuous basis. It isn’t just light waves that travel through the Universe, nor is that light merely joined by gravitational waves and ghostly neutrinos. In truth, cosmic particles and antiparticles of all types are produced in high-energy processes throughout the Universe, from the Big Bang to stars to white dwarfs to neutron stars to black holes, both large and small. When we put detectors up to detect what sorts of particles are out there, we find a virtual zoo, including:
- protons,
- antiprotons,
- electrons,
- positrons,
- and even still-heavier atomic nuclei, made out of protons and neutrons combined.
Most cosmic rays, as we measure them, turn out to be protons, and just as you’d expect, there are more of them at lower energies and fewer and fewer of them as you look to ever-higher energies. However, there’s a theoretical limit to how energetic even the highest-energy cosmic rays ought to be: about 50 exa-electron-volts (50 EeV, or 5 × 1019 electron-volts), a limit known as the GZK cutoff. In 1991, the Fly’s Eye camera in Utah observed a particle so energetic it baffled astrophysicists, who termed the 320 EeV particle the Oh-My-God particle. 30 years later, in 2021, we saw our second particle of comparably large energies, with the Telescope Array Collaboration observing a 240 EeV particle.
Although it’s easy to find headlines about scientists being baffled by such particles, the sober truth is we have an excellent idea of what’s going on. Here’s the simplest explanation, which some might even dare to call “ironic.”
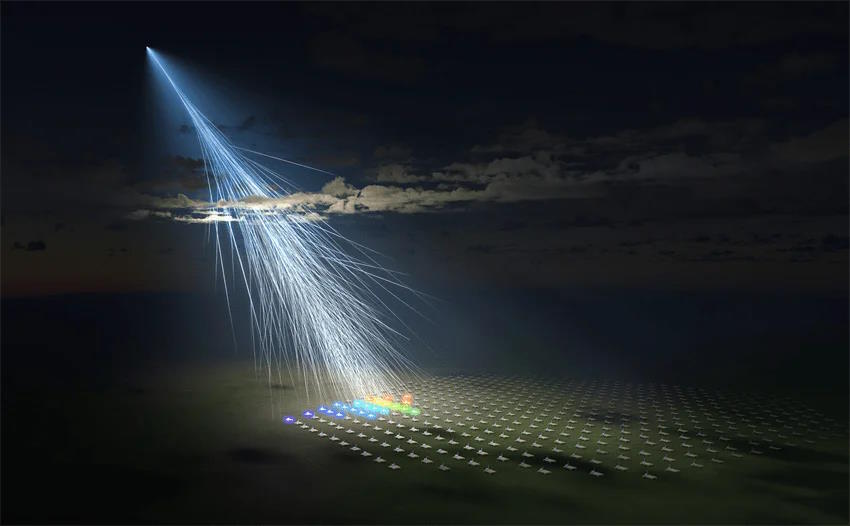
The science, and census, of cosmic rays
When it comes to the generation of cosmic rays, there are three things you have to always consider.
- There are energetic places in the Universe: where large numbers of particles are gathered together in one place, creating a massive, usually hot set of conditions. These places can be around objects like stars, stellar corpses, and even active black holes of all masses, from the stellar mass type all the way up to supermassive ones.
- These energetic places are always in motion, often very rapidly, both internally and relative to one another.
- And furthermore, these energetic places that are in motion, either made up of charged particles (including protons and electrons) or that are surrounded by matter that’s composed of charged particles (such as the environments around active black holes), will generate both electric and magnetic fields which can be very strong.
That recipe, with lots of mass together in one place, with rapid, often relativistic (near the speed-of-light) motions, possessing incredibly strong electric and magnetic fields, is exactly what you need to create cosmic rays: individual particles that are emitted from their source and accelerated to great speeds, speeds that will send them hurtling all across the Universe.
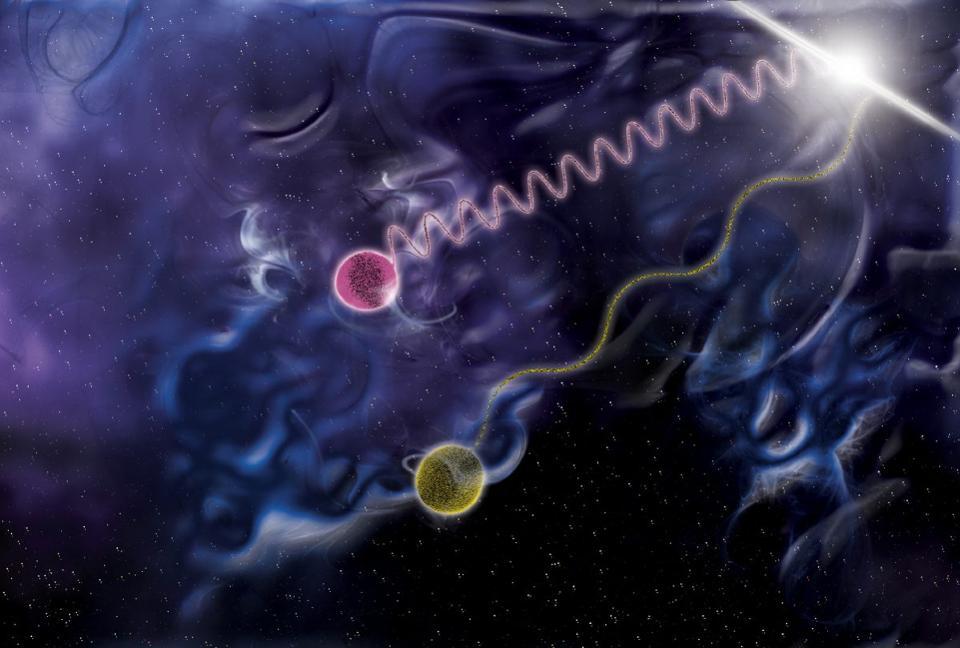
We’ve developed a great many instruments and techniques to measure and count these cosmic rays, uncovering a wide variety of their properties. The science of cosmic rays began more than 100 years ago, when scientists began bringing particle detectors and tracking devices/chambers up to very high altitudes on hot air balloon rides, where they would detect exotic particles that could only have been produced from high-energy particle-particle collision in the upper atmosphere: where particles traveling through space first collide with Earth’s most tenuously held atmospheric layers.
This has developed into a spectacularly modern scientific endeavor, with large, precise detectors aboard missions in space, with ground-based telescopes and enormous arrays of telescopes that look for particle signatures of cosmic ray showers (then using that data to reconstruct information about the particle that first struck Earth’s atmosphere to create these showers), and with telescopes that look for the ultraviolet light produced by very fast-moving particles that stream through our atmosphere with speeds greater than the speed of light (in the medium of our atmosphere, not in a vacuum).
We’ve found that most cosmic rays are at modest energies, with very high energy cosmic rays existing but only in much smaller numbers, and that most cosmic rays are protons, with a small fraction being electrons or positrons, another small fraction being antiprotons, but with the remainder being composed of heavier atomic nuclei: helium, carbon, and so on, all the way up to the nuclei of elements like iron.
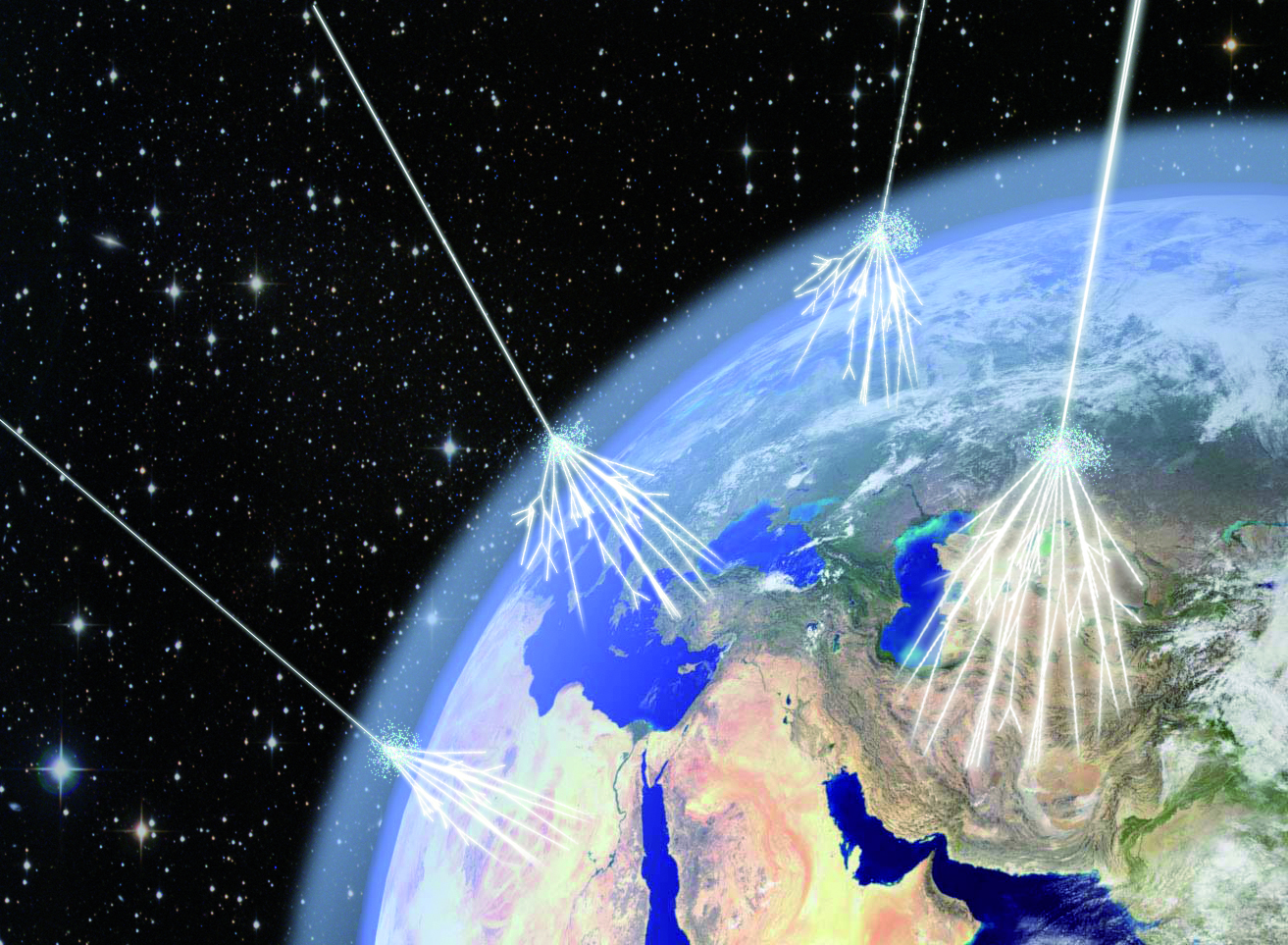
The extremes of energies
You might think that particles, once created, simply travel through the vacuum of space until they reach our detectors. (Or, at least, until they reach the top of Earth’s atmosphere.) However, there are good reasons to expect that simply by traveling through the Universe, the highest-energy particles will rapidly lose energy until they drop below a critical threshold. The biggest reason, which might come as a surprise is simply this: space isn’t empty, but rather is full of “stuff,” including particles, neutrinos and antineutrinos, and photons.
If we count them all up, we might add “especially photons,” as there are an estimated 411 photons occupying every cubic centimeter of even deep, intergalactic space, mostly left over from the Big Bang. All told, all throughout the observable Universe, there are somewhere around a total of ~1089 photons present: the most abundant and also the most evenly distributed type of quantum present within our known Universe.
And, if you think about what cosmic rays are made out of — protons, antiprotons, electrons, positrons, and heavy atomic nuclei — you’ll realize they’re all electrically charged. This is important, as there’s always a significant probability for a charged particle and a photon, regardless of what the relative energies of the particle and the photon are, to interact.
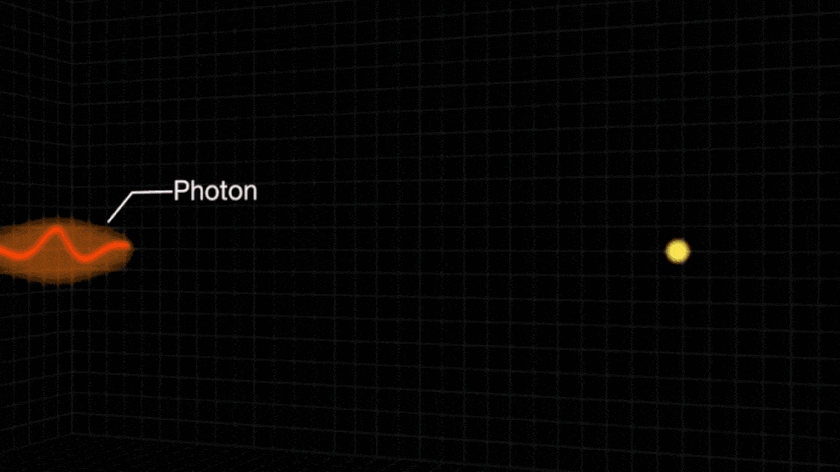
At low energies, this is boring and just results in the transfer of energy and momentum: Thomson scattering. At higher energies, this is only slightly less boring and results in the transfer of energy and momentum but with relativity included: Compton scattering. But at extremely high energies, such as those achieved by the highest energy cosmic rays, things get very interesting. When photons and charged particles collide, they not only scatter, transferring energy and momentum, but if enough energy is available, they can create new particles (and antiparticles) through Einstein’s most famous equation: E = mc².
The fastest protons created in the laboratory are made at the Large Hadron Collider here on Earth. They can reach speeds of 299,792,455 m/s (just ~3 m/s slower than the speed of light), which corresponds to energies of up to 7 TeV (or 7 × 1012 eV), and if a proton of these energies runs into a leftover photon from the Big Bang (with an average energy of only around 0.0002 eV), there isn’t enough combined energy to create any new particles or antiparticles.
But at tens of thousands of times that energy, starting at around ~1017 eV, now something different occurs.

When protons at or above that much higher energy threshold now collide with photons left over from the Big Bang (i.e., CMB photons), there’s enough energy to spontaneously produce electron-positron pairs. If a proton only behaves as though it’s scattering off of a photon, it’s no big deal, as those interactions cause the proton to lose only a negligible amount of energy: less than a millionth-of-a-percent per interaction. But if it behaves as though it’s scattering off of either an electron or a positron — which is what it experiences at energies of ~1017 eV and up — each one of those collisions will drain about 0.1% of the original proton’s energy. Over the millions of light-years that separate galaxies from one another, that energy loss from a large number of interactions can really add up.
But the next threshold up, at still higher energies, is much more severely limiting. When there suddenly becomes enough energy to spontaneously produce a subatomic particle known as a neutral pion (π0) from a proton-photon collision via Einstein’s E = mc², the original proton’s energy gets nerfed by a very large amount: reducing it by about 20% with each such interaction. After traveling for just ~100–200 million years through the intergalactic medium — a blip in time compared to the 13.8 billion year age of the Universe — all protons should fall below that limiting energy threshold: around 5 × 1019 eV. That threshold is known as the GZK cutoff, after its computation in 1966 by Kenneth Greisen, Georgiy Zatsepin, and Vadim Kuzmin. Unlike protons at the Large Hadron Collider that reach a speed of 99.999999% the speed of light, the GZK cutoff should cap out the speeds of protons at a much greater speed: 99.99999999999999999998% the speed of light: about ~60 femtometers-per-second below the ultimate cosmic limit.
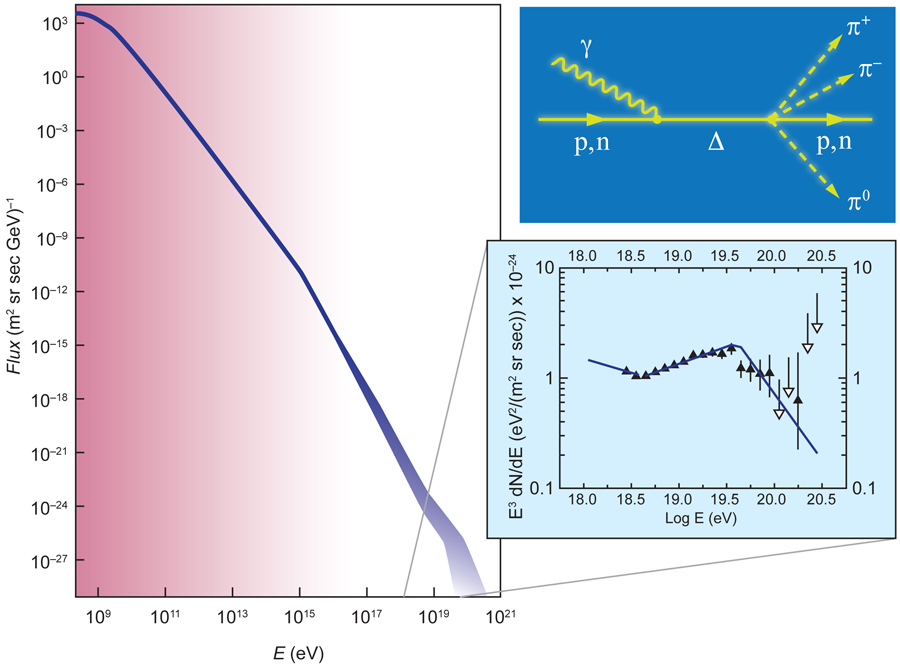
Surpassing conventional limits
Of course, that theoretical cutoff doesn’t correspond to the actual observed limit of cosmic rays. There’s a steady decline in how many there are of a given energy down to about the predicted GZK cutoff, and then there’s a further decline in how many there are, but not a rapid drop to zero. In fact, there have now been hundreds of events measured to exceed the GZK cutoff, rising as high as ~7 times the supposed maximum energy that cosmic rays should be observed to have.
Moreover, they are not associated with known high-energy sources of cosmic particles, including:
- identified neutron stars,
- known galactic black holes,
- supermassive black holes,
- active galactic nuclei or quasars,
- or even distant galaxies.
Instead, they appear to be neither clumped nor clustered together, and they appear to come from random, uncorrelated directions. For a long time, there were many scientists who hoped that 1991’s Oh-My-God particle and other high-energy particles measured with that same, old instrument, would simply turn out to be a measurement anomaly. The hope was that subsequent, superior detector arrays — such as the Pierre Auger Observatory and the Telescope Array — would bring observations back in line with the GZK cutoff’s predictions.
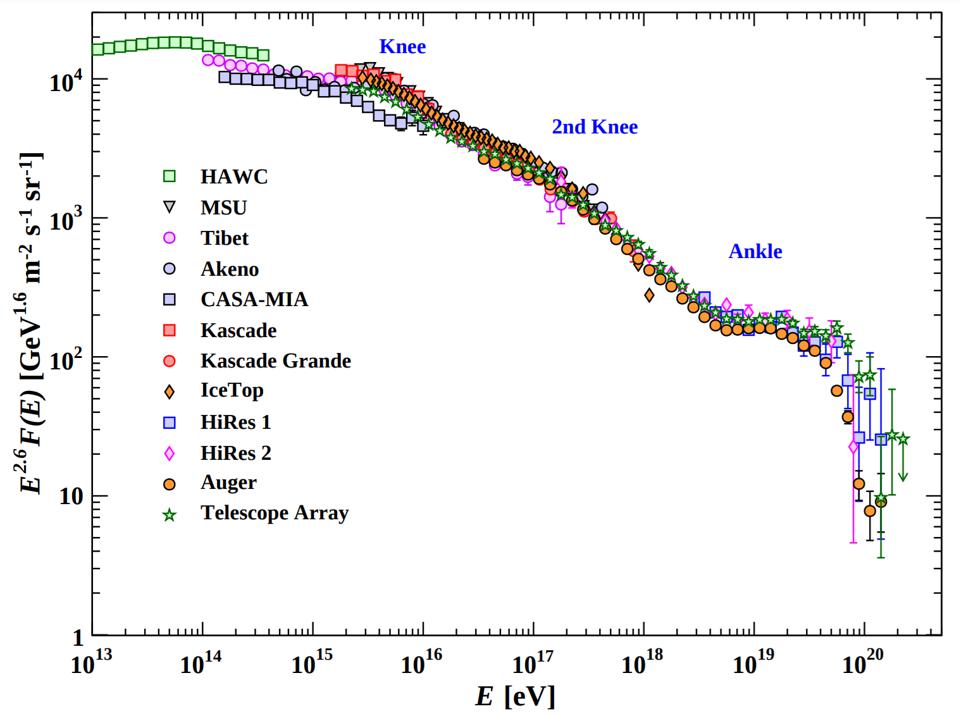
They have not. Instead, we’re faced with a reality where 50 EeV isn’t an energy cutoff at all, but where particles exist in copious numbers up above that: with dozens of particles above 100 EeV, and the recent Amaterasu (translated as “Sun Goddess”) particle reaching 240 EeV, the second-highest of all-time behind 1991’s 320 EeV Oh-My-God particle. If you listen to the words of the particle’s discoverer, Toshihiro Fujii, you might mistakenly think that particles such as this are complete mysteries.
“When I first discovered this ultra-high-energy cosmic ray, I thought there must have been a mistake, as it showed an energy level unprecedented in the last three decades.”
Telescope Array Collaboration member John Belz had no problem speculating wildly about the possible origins of these extreme energy cosmic rays.
“It could be defects in the structure of spacetime, colliding cosmic strings. I mean, I’m just spitballing crazy ideas that people are coming up with because there’s not a conventional explanation.”
But there’s a super simple explanation that, for some reason, always gets ignored: perhaps the reason these particles are reaching such great energies is because they aren’t protons, but rarer, heavier atomic nuclei. With greater masses than a proton (an iron nucleus is ~56 times a proton’s mass), the GZK cutoff corresponds to the same speed for those heavier nuclei, but admits greater masses and, in turn, greater energies.
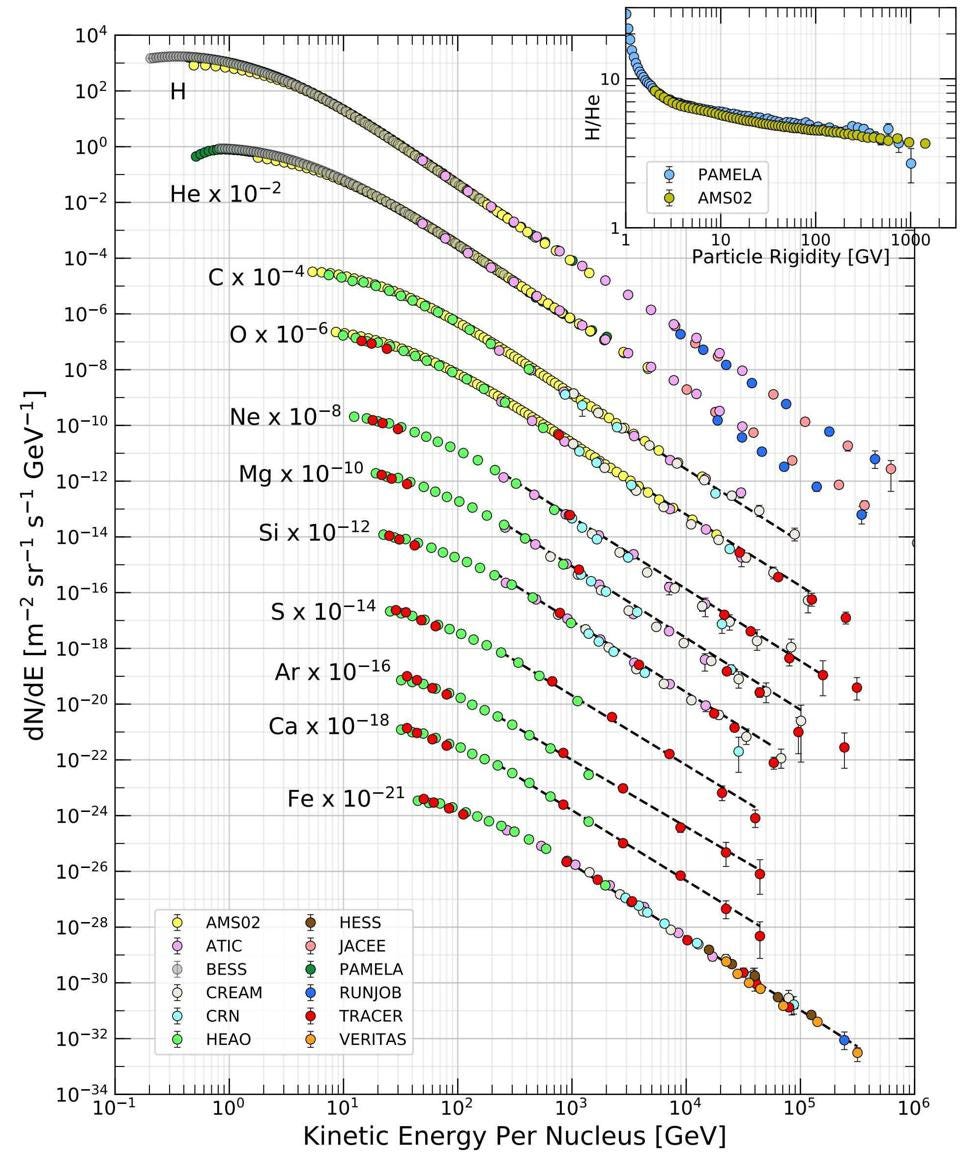
The simple explanation and a continuing mystery
It turns out that while around 85% of cosmic rays are protons and about 10% are alpha particles (helium nuclei), about 1% of them are what are called HZE ions, or high atomic number and energy particles. Notably, these include carbon, oxygen, magnesium, silicon, calcium, and iron, among others. They are found in cosmic rays from the Sun, in cosmic rays from within the Milky Way, and even among (although less abundantly) the extragalactic cosmic rays. While the GZK cutoff applies to single protons, these extreme-energy cosmic rays, the ones above the GZK cutoff, might exclusively be composed of heavier nuclei: helium, carbon, oxygen, iron, and so on.
In other words, you don’t need an exotic explanation to account for these. Rather than invoking an idea like:
- relativity is wrong,
- these high energy particles all come from sources within our own Milky Way (so that they don’t have the time/opportunity to drop below the GZK limit),
- or there’s some sort of ultra-high-energy cosmic accelerator called a Zevatron that we just haven’t discovered yet,
it could simply be that the highest-energy cosmic rays are made out of atomic nuclei that are heavier than single protons, meaning that there’s nothing more “extreme” about these particles than the other ultra-high-energy cosmic rays that fall below the GZK cutoff.
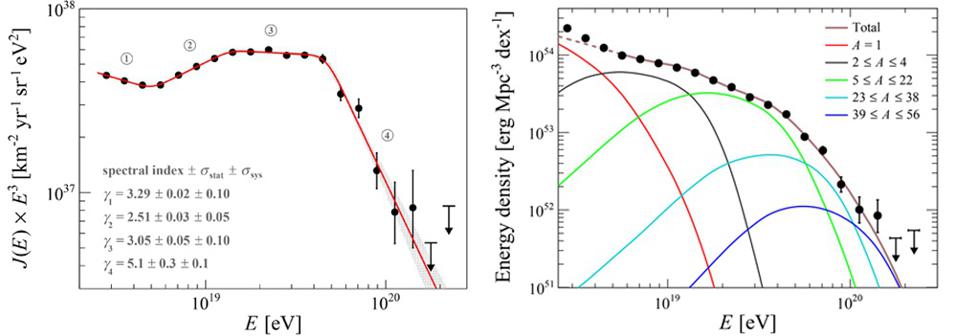
In other words, the simplest explanation for these particles is simply that they’re heavier atomic nuclei, and that so long as each individual proton-and-neutron within that nucleus doesn’t exceed the GZK cutoff for energy, collisions with CMB photons won’t spontaneously produce pions and won’t spontaneously bring down the energies of these cosmic rays fast enough. That ought to be the null hypothesis: the simplest explanation that’s consistent with everything we know, expect, and also observe.
However, there is still a mystery that endures, not only for these extreme-energy particles but practically all of the ultra-high-energy cosmic rays: we don’t know what causes them. The more modern observatories can pinpoint the directions from which these cosmic rays originate, and have determined that they’re not correlated with any particular set of directions in the sky. They’re not correlated with features within our own galaxy, nor neutron stars, nor active supermassive black holes, nor supernovae, nor any other identifiable features. We know that these cosmic rays are out there, and we strongly suspect they have a conventional astrophysical cause. But we don’t actually know what that cause, or the mechanism behind the energies of these particles, happens to be.
It’s a remarkable find and a fun puzzle, but scientists are no more baffled about cosmic rays after the discovery of this Amaterasu particle than they were before. The simplest explanation — that it was simply a heavy ion traveling at high speeds throughout the Universe before arriving at Earth — still checks all the boxes.