The Death of Dark Matter’s #1 Competitor
The only way out is to modify the laws of gravity, and new constraints rule those modifications out.
“The discrepancy between what was expected and what has been observed has grown over the years, and we’re straining harder and harder to fill the gap.” –Jeremiah P. Ostriker
If you have any sort of interest in outer space, the Universe and just what this entire existence is made up of, you’ve probably heard of dark matter — or at least the dark matter problem — before. In brief, let’s take a look at what you might see if you looked out at the Universe with the greatest telescope technology we’ve ever developed as a species.
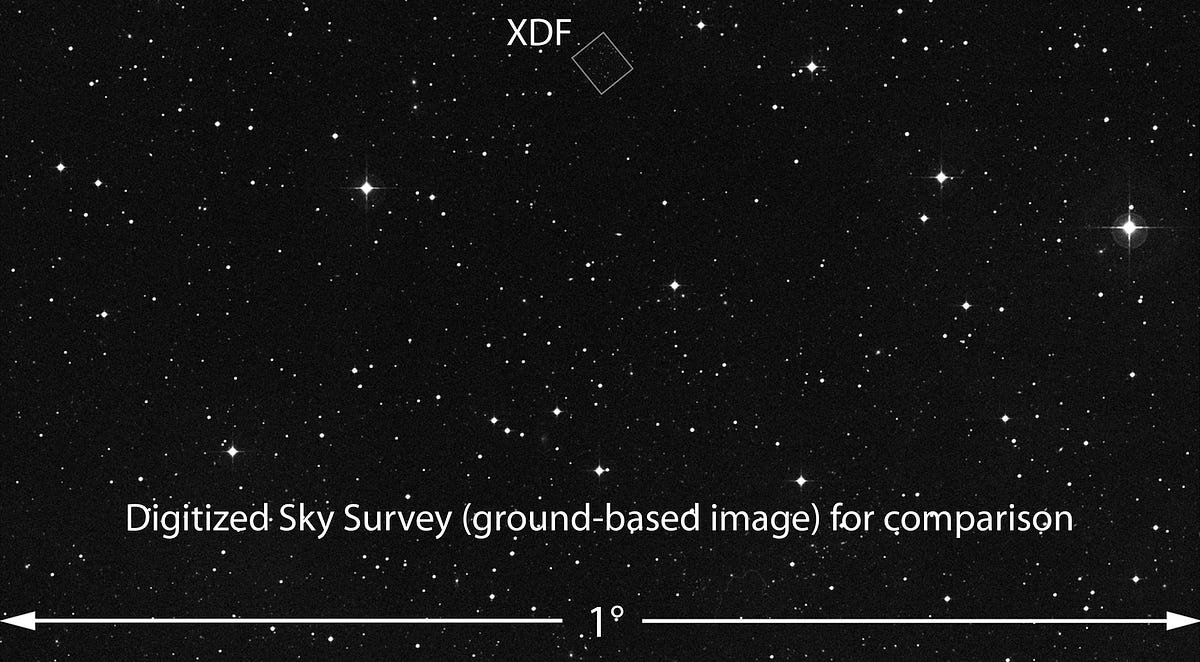
Not this image, of course. This is what you’d see to the significantly aided human eye: a small region of space that contains just a handful of dim, faint stars present within our own galaxy, and apparently nothing beyond it.
What we’ve done is look at not only this region in particular, but many others like it, with incredibly sensitive instruments. Even in a region like this, devoid of bright stars, galaxies, or known clusters or groups, all we have to do is point our cameras at it for arbitrarily long stretches of time. If we let enough go by, we start to collect photons from incredibly faint, distant sources. That tiny box marked “XDF” above is the location of the Hubble eXtreme Deep Field, a region so small it would take 32,000,000 of them to cover the entire night sky. And yet, here’s what Hubble saw.
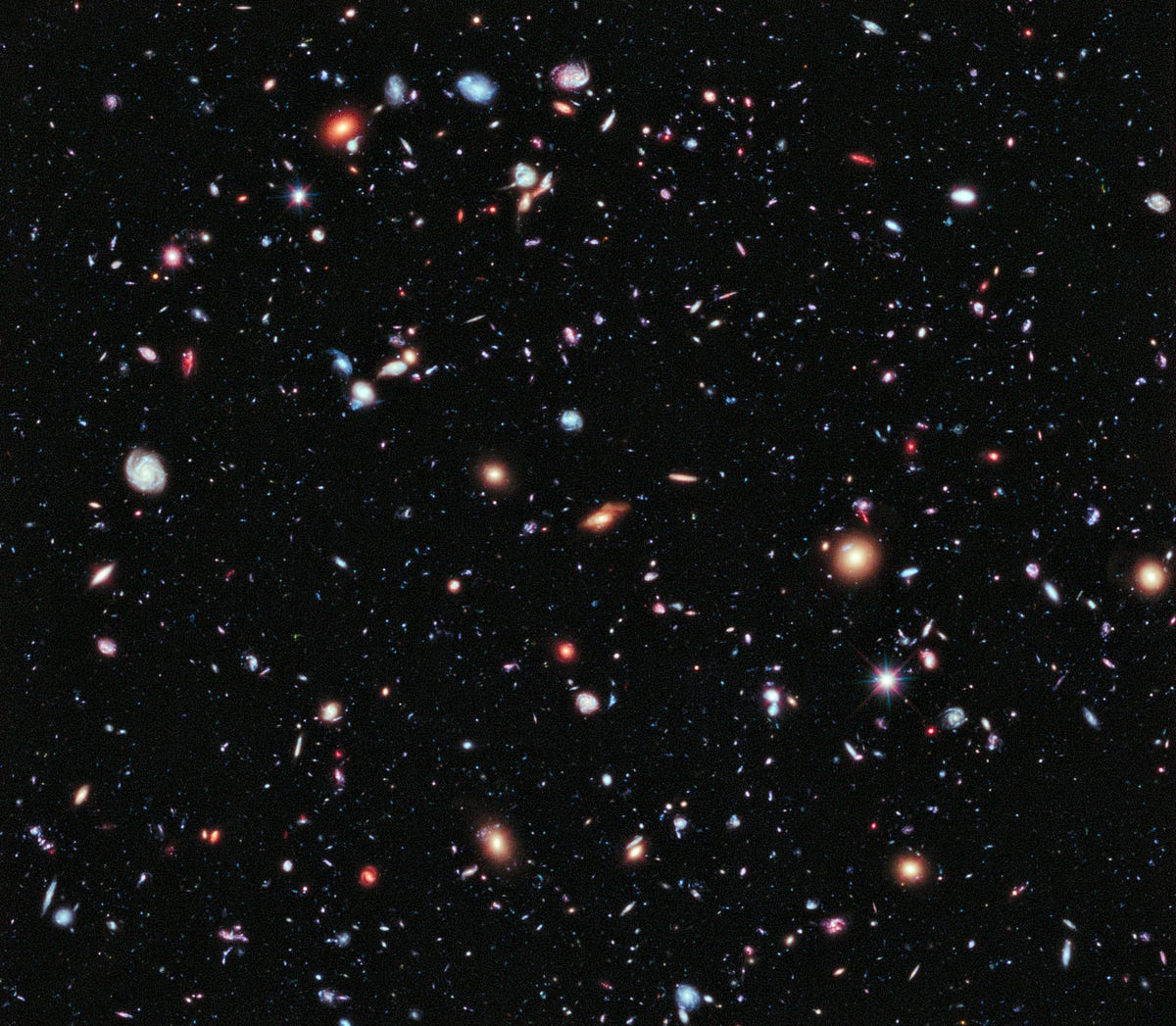
There are 5,500 unique galaxies identified in this image, meaning that there are at least 200 billion galaxies in the entire Universe. But as impressive as that number is, it’s not even the most impressive thing we’ve learned about the Universe from studying the huge number and diversity of galaxies, groups and clusters within it.
Think about what’s making these galaxies shine, whether right next door to us or tens of billions of light-years away.
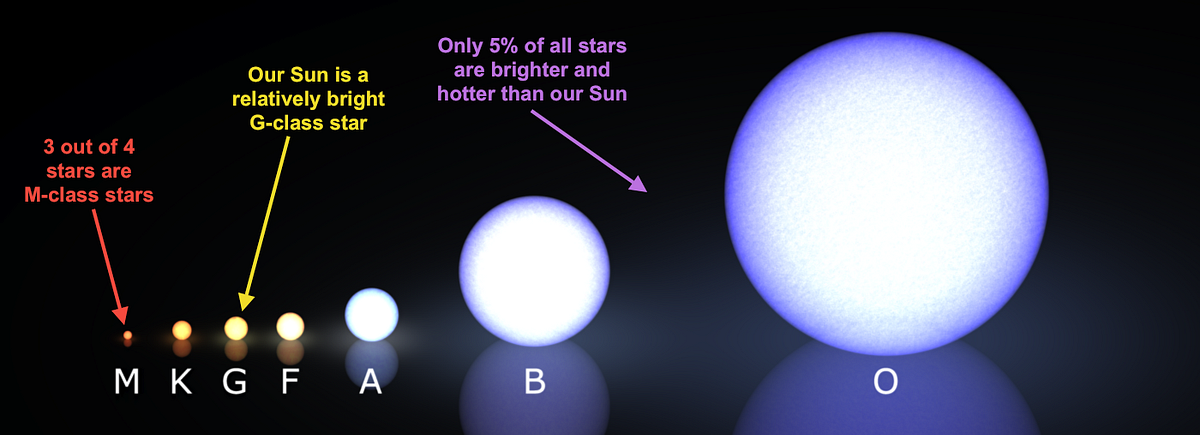
It’s the stars shining within them! Over the past 150 years or so, one of the greatest achievements of astronomy and astrophysics has been our understanding of how stars form, live, die, and shine while they’re alive. When we measure the starlight coming from any one of these galaxies, we can immediately infer exactly how what types of stars are present within it, and what the total mass of the stars inside is.
Hold this in your mind as we move forward: the light we observe from the galaxies, groups and clusters we see tells us how much mass is in that galaxy’s, group’s or cluster’s stars. But starlight isn’t the only thing we can measure!
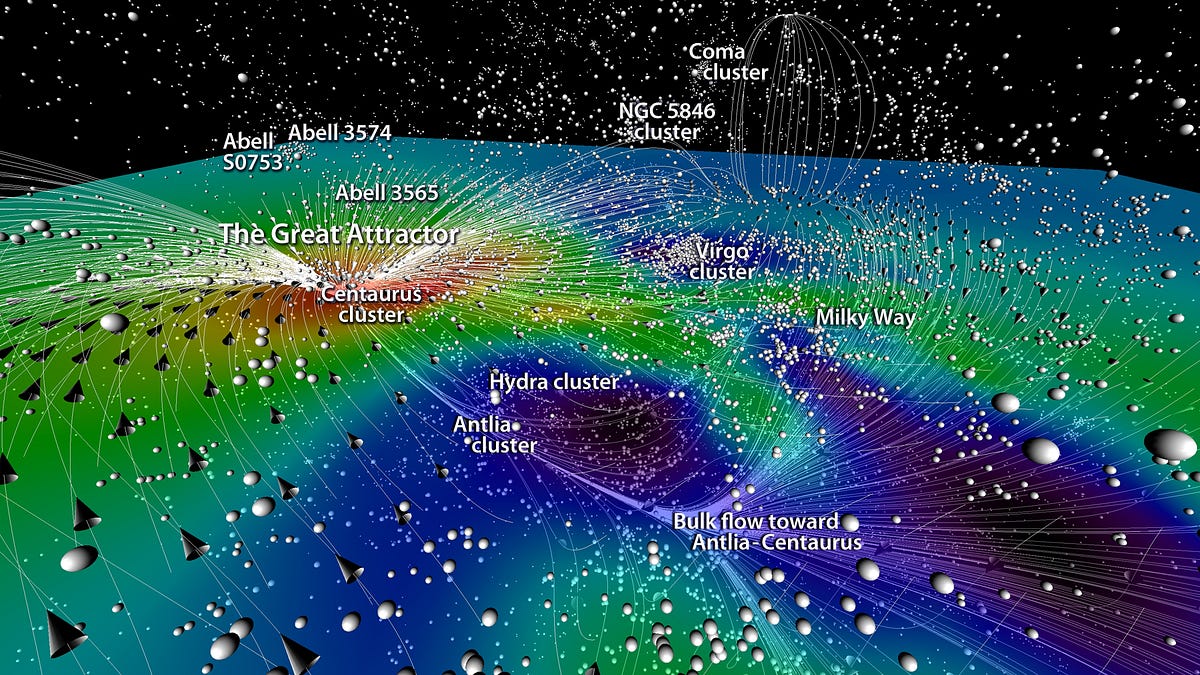
We can also measure how these galaxies are moving, how quickly they’re rotating, what their velocities are relative to one another, and so on. This is incredibly powerful, because based on the laws of gravity, if we measure the velocities of these objects, we can infer how much mass-and-matter there must be inside of them!
Think about that for a moment: the law of gravitation is universal, meaning it’s the same everywhere in the Universe. The law that governs the Solar System must be the same as the law that governs the galaxies. And so here we have two different ways of measuring the mass of the largest structures in the Universe:
- We can measure the starlight coming from them, and because we know how stars work, we can infer how much mass there is in stars in these objects.
- We can measure how they’re moving, knowing whether-and-how they’re gravitationally bound. From gravitation, we can infer how much total mass there is in these objects.
So now we ask the crucial question: do these two numbers match?
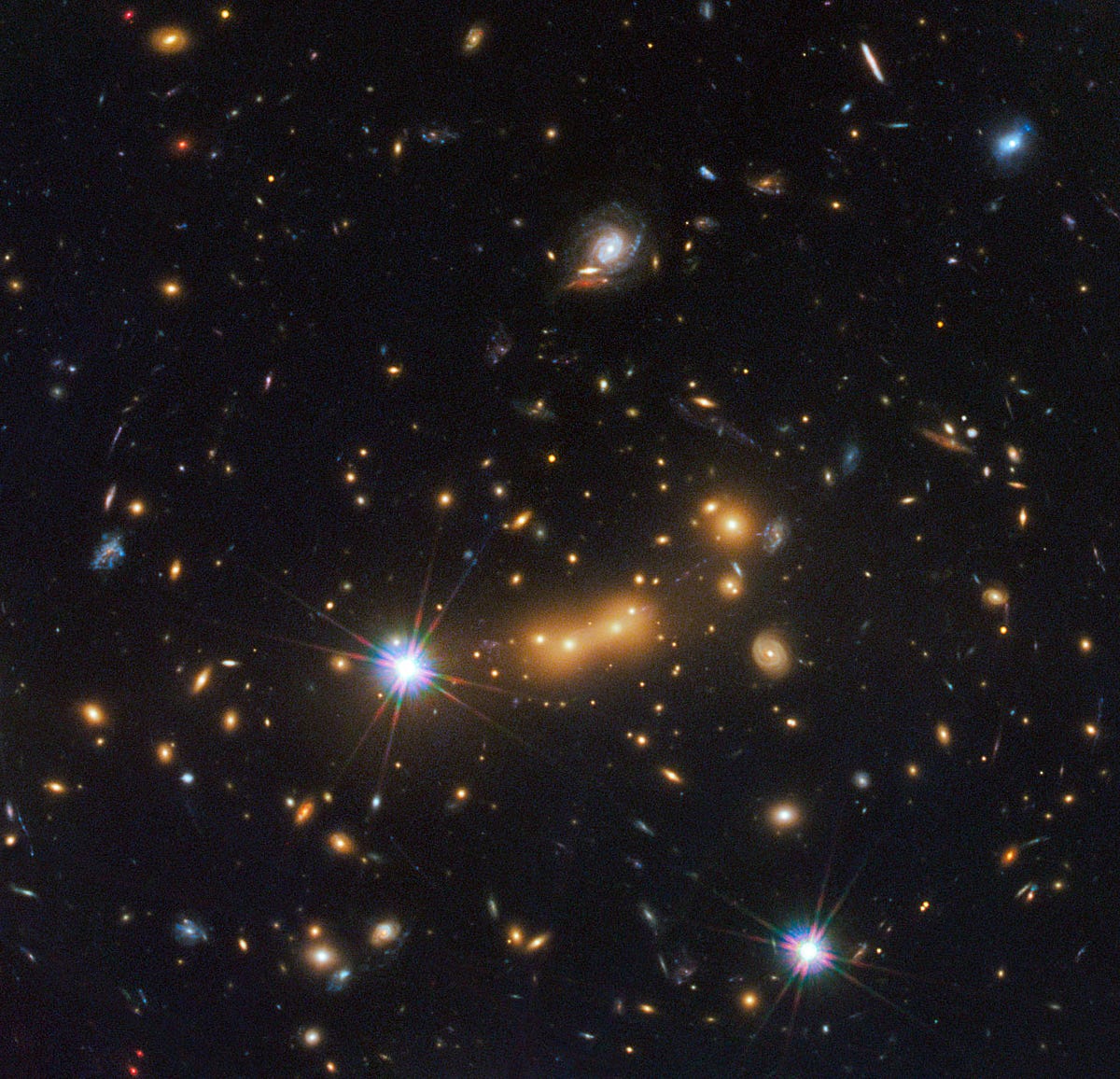
Not only do they not match, they’re not even close! If you compute the amount of mass present in stars, you get a number, and if you compute the amount of mass that gravitation tells us must be there, you get a number that’s 50 times greater. This is true regardless of whether you look at small galaxies, large galaxies or groups or clusters of galaxies.
Well, that tells us something important: either whatever’s making up 98% of the mass of the Universe isn’t stars, or our understanding of gravitation is wrong. Let’s take a look at the first option, because we have a lot of data there.
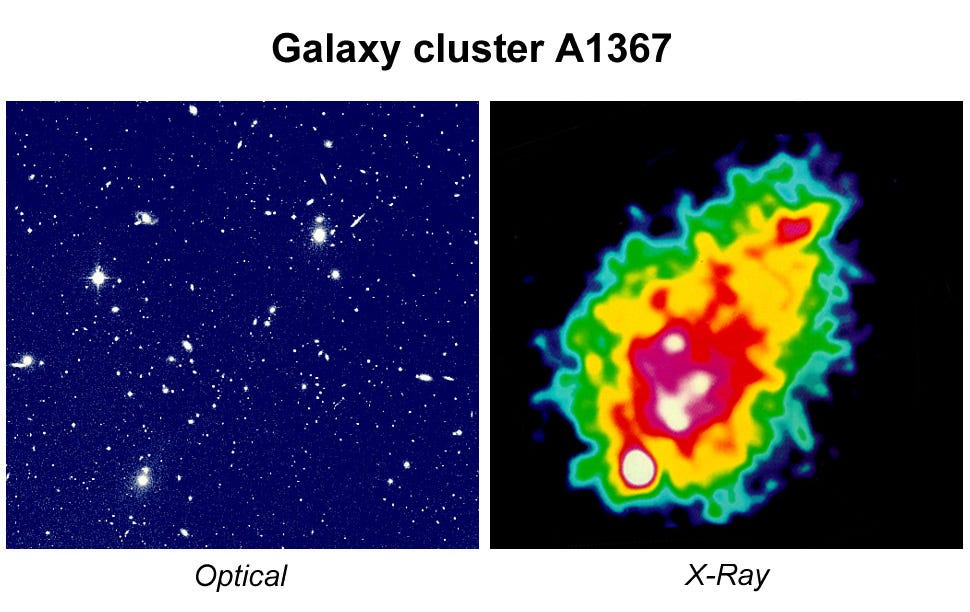
There could be lots of other things out there besides stars that make up the mass of galaxies and clusters, including:
- clumps of non-luminous matter like planets, moons, moonlets, asteroids, iceballs, etc.,
- neutral and ionized interstellar gas, dust, and plasma,
- black holes,
- stellar remnants like white dwarfs and neutron stars
- and very dim stars or dwarf stars.
The thing is, we’ve measured the abundance of these objects and — in fact — the total amount of normal (i.e., made of protons, neutrons and electrons) matter in the Universe from a variety of independent lines, including the abundance of the light elements, the cosmic microwave background, the large scale structure of the Universe, and from astrophysical surveys. We’ve even tightly constrained the contribution of neutrinos; here’s what we’ve learned.
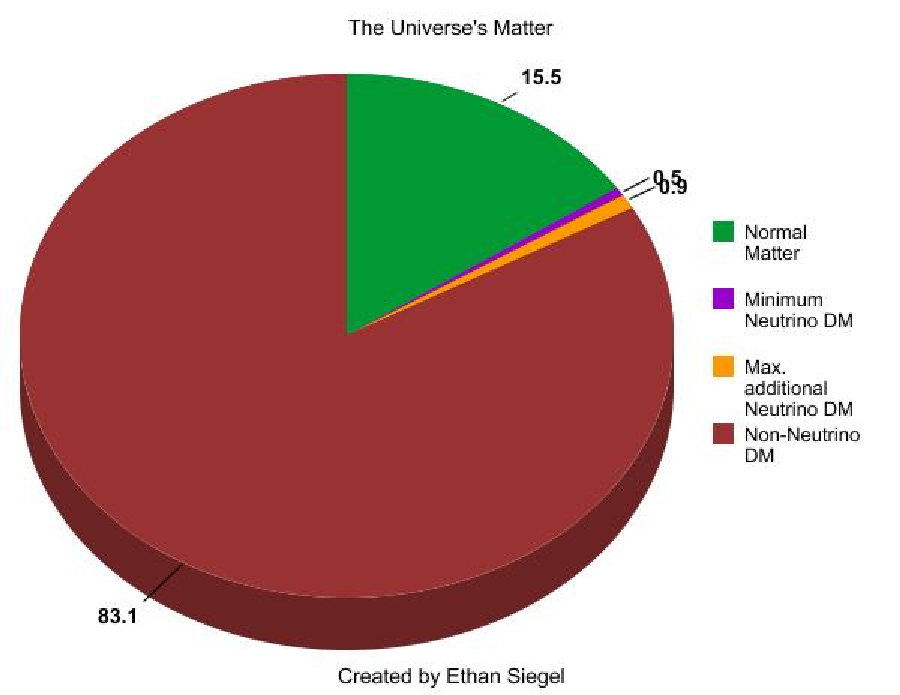
About 15-16% of the total amount of matter in the Universe is made up of protons, neutrons and electrons, the majority of which is in interstellar (or intergalactic) gas and plasma. There’s maybe about another 1% in the form of neutrinos, and the rest must be some type of mass that’s not made up of any particles present in the Standard Model.
That’s the dark matter problem. But it’s possible that postulating some unseen, new form of matter isn’t the solution, but that the laws of gravity on the largest scales are simply wrong. Let me walk you through a brief history of the dark matter problem, and what we’ve learned about it as time has gone on.
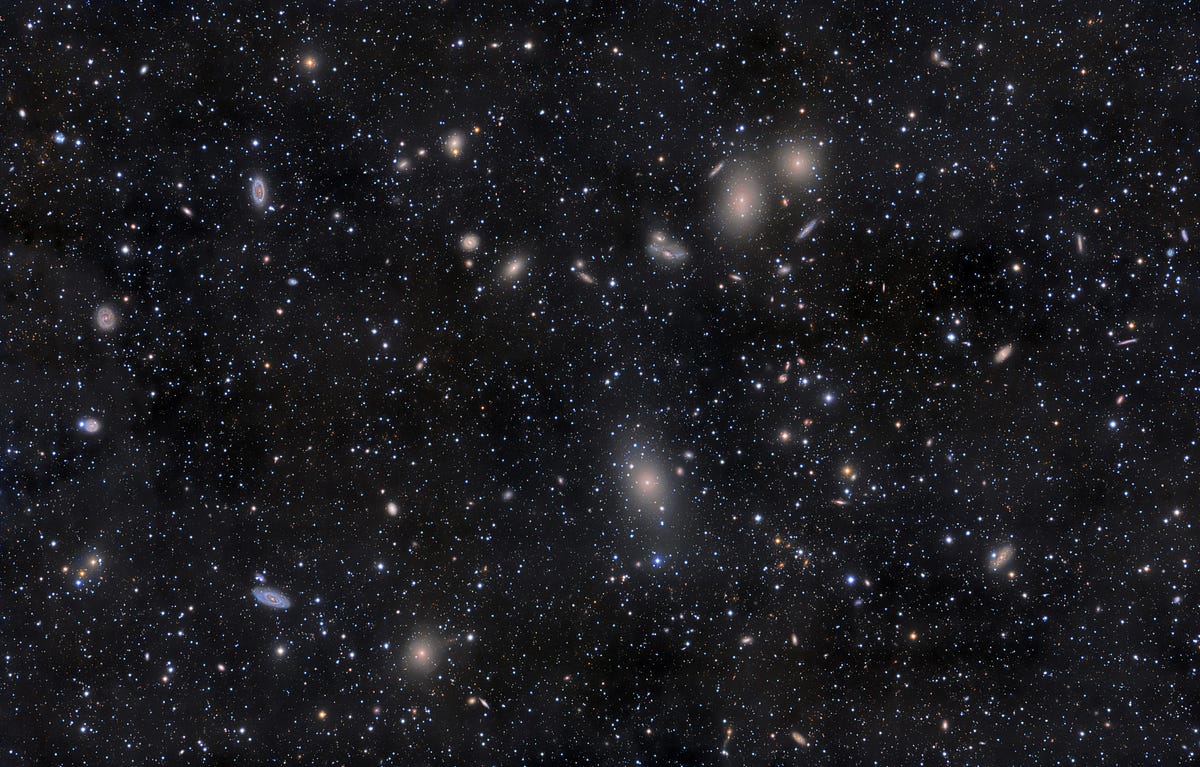
Large-scale structure formation — at least initially — was poorly understood. But starting in the 1930s, Fritz Zwicky began measuring the starlight coming from galaxies present in clusters, as well as how quickly the individual galaxies were moving relative to one another. He noted the huge discrepancy mentioned above between the mass present in stars and the mass that must be present to keep these large clusters bound to one another.
This work went largely ignored for about 40 years.
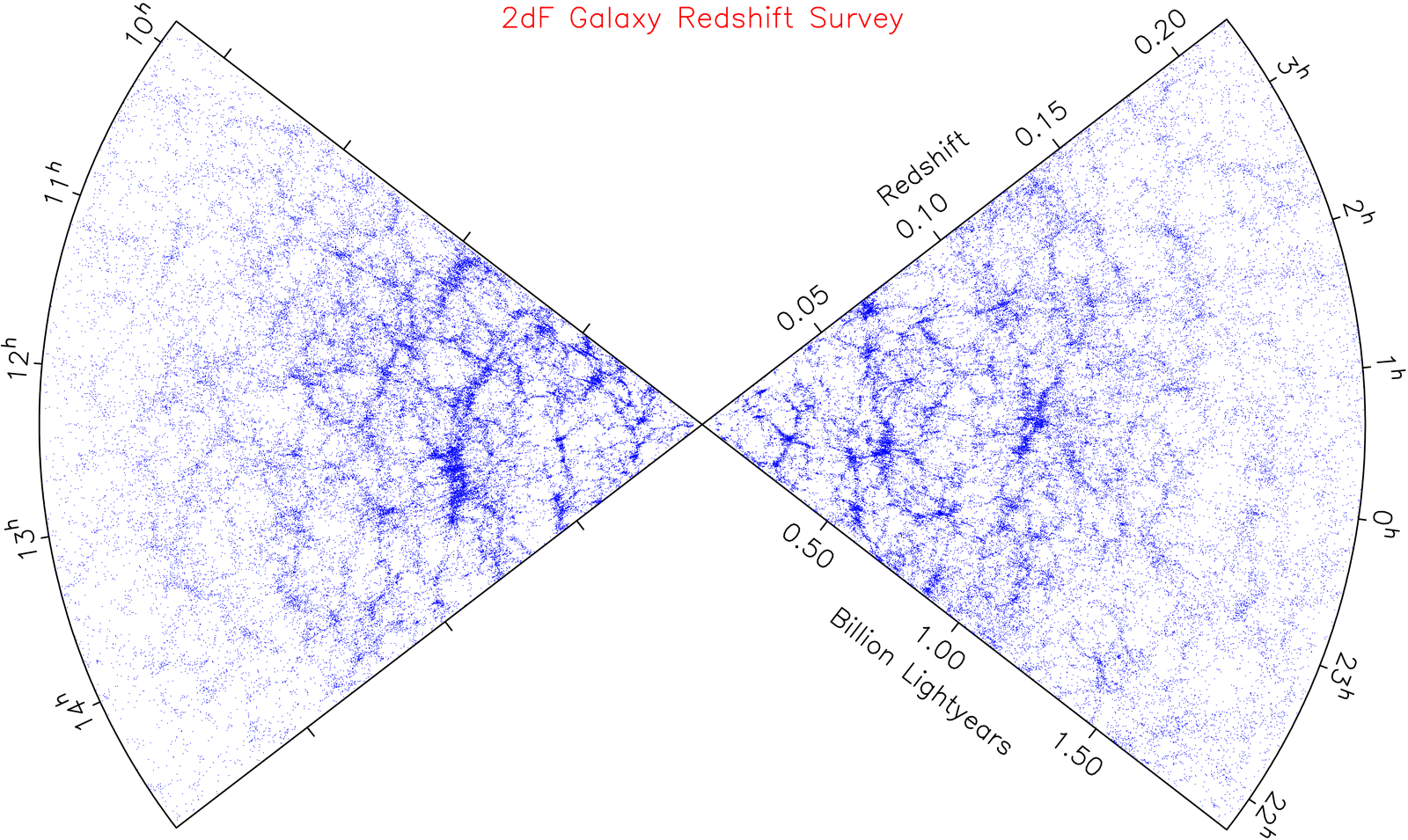
When we started making large cosmological surveys in the 1970s, such as PSCz, their results started to indicate that in addition to Zwicky’s cluster-dynamics problems, the structure we were seeing on even larger-scales required an unseen, non-baryonic source of mass to reproduce the structures observed. (This has since been improved by surveys like 2dF, above, and SDSS.)
Also in the 1970s, Vera Rubin’s original and hugely influential work brought new attention to rotating galaxies, and the dark matter problem they showcased so thoroughly.
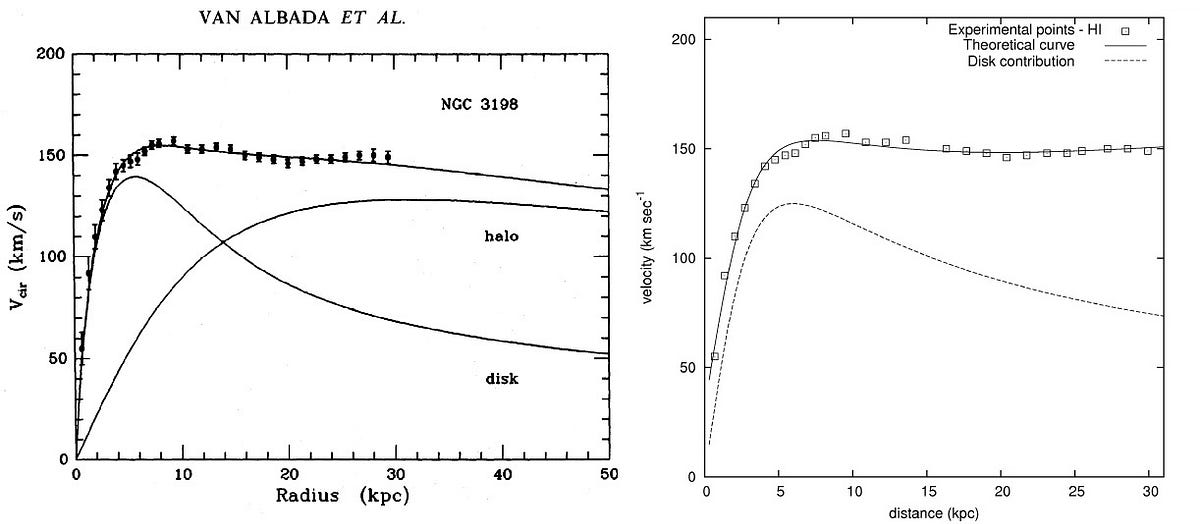
Based on what was known about the law of gravity and what was observed about the density of normal matter in galaxies, you would have expected that as you moved farther away from the center of a spinning, spiral galaxy, the stars orbiting it would slow down. This should be very similar to the phenomenon seen in the Solar System, where Mercury has the highest orbital speed, followed by Venus, then by Earth, then by Mars, etc. But what spinning galaxies show instead is that the rotational speed appears to remain constant as you move out to larger and larger distances, which tells us that either there’s more mass than can be accounted for by normal matter, or that the law of gravity needs to be modified.
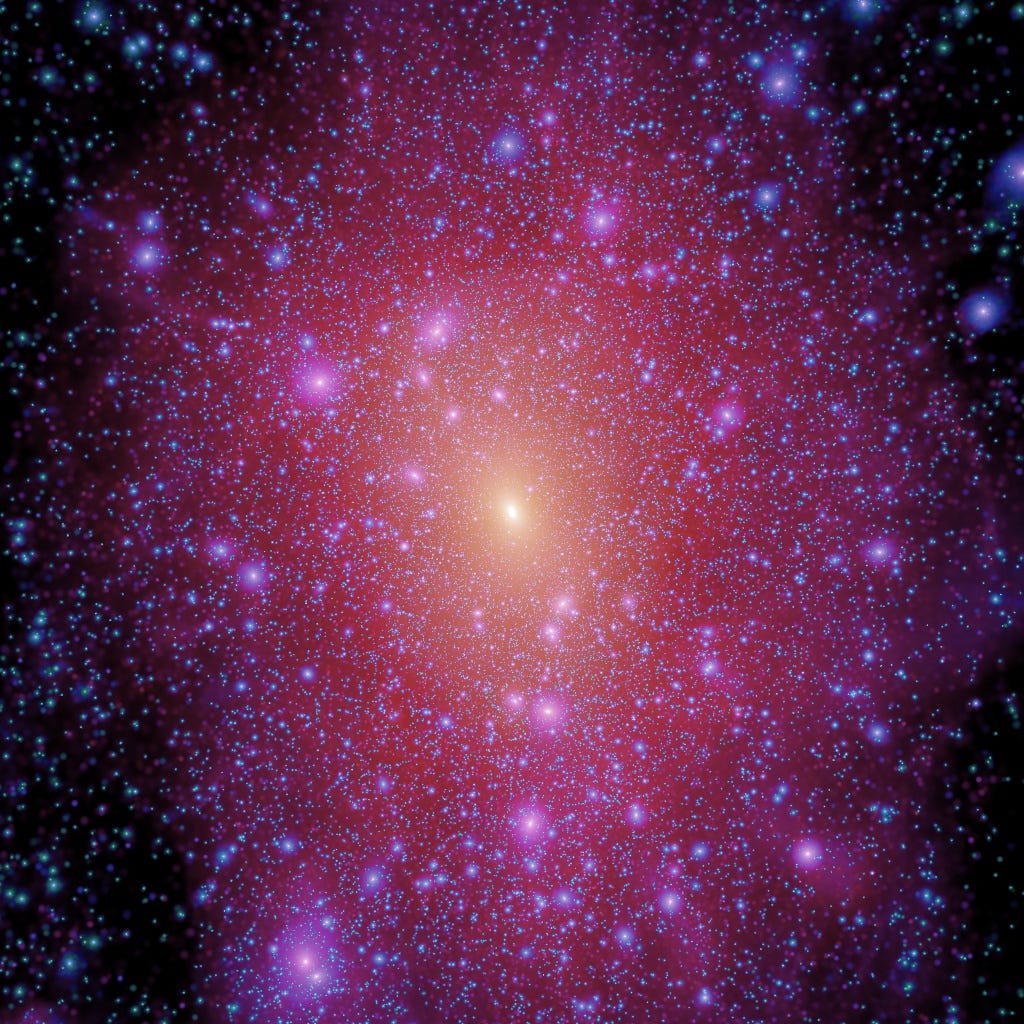
Dark matter was the leading proposed solution to these problems, but no one knew whether it was all baryonic or not, what its temperature properties were, and whether/how it interacted with both normal matter and itself. We had some limits and constraints on what it couldn’t do, and some early simulations that seemed promising, but nothing concretely convincing. And then the first major alternative came along.
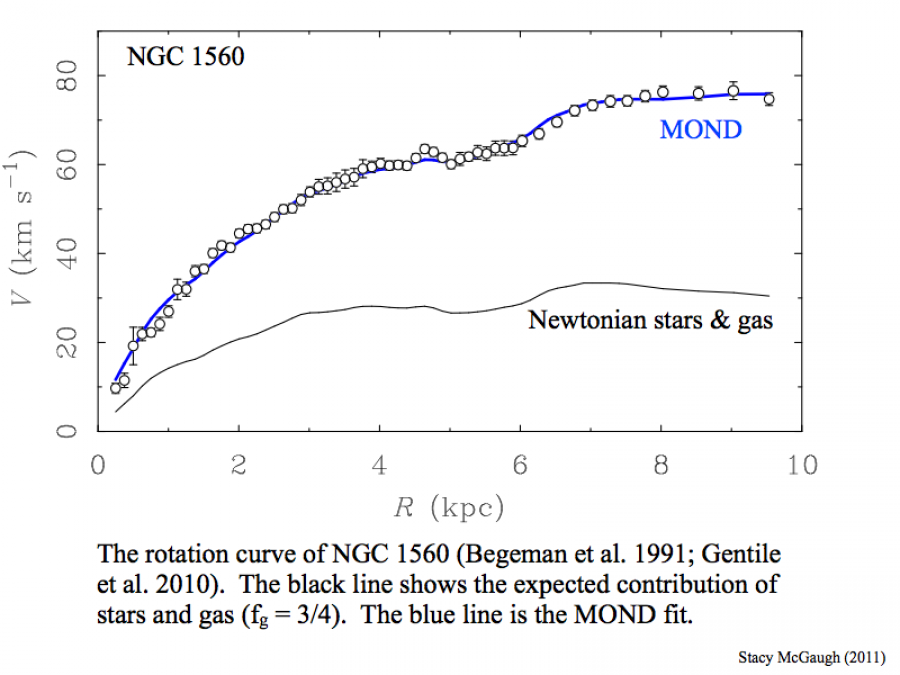
MOND — short for MOdified Newtonian Dynamics — was proposed in the early 1980s as a phenomenological, empirical fit to explain the rotating galaxies. It worked very well for small-scale structure (galaxy-scale), but failed on large scales in all models. It couldn’t explain galaxy clusters, it couldn’t explain large-scale structure, and it couldn’t explain the abundance of the light elements, among others.
While the galaxy dynamics people latched on to MOND because it is more successful at predicting galactic rotation curves than dark matter is, everyone else was highly skeptical, and for good reason.
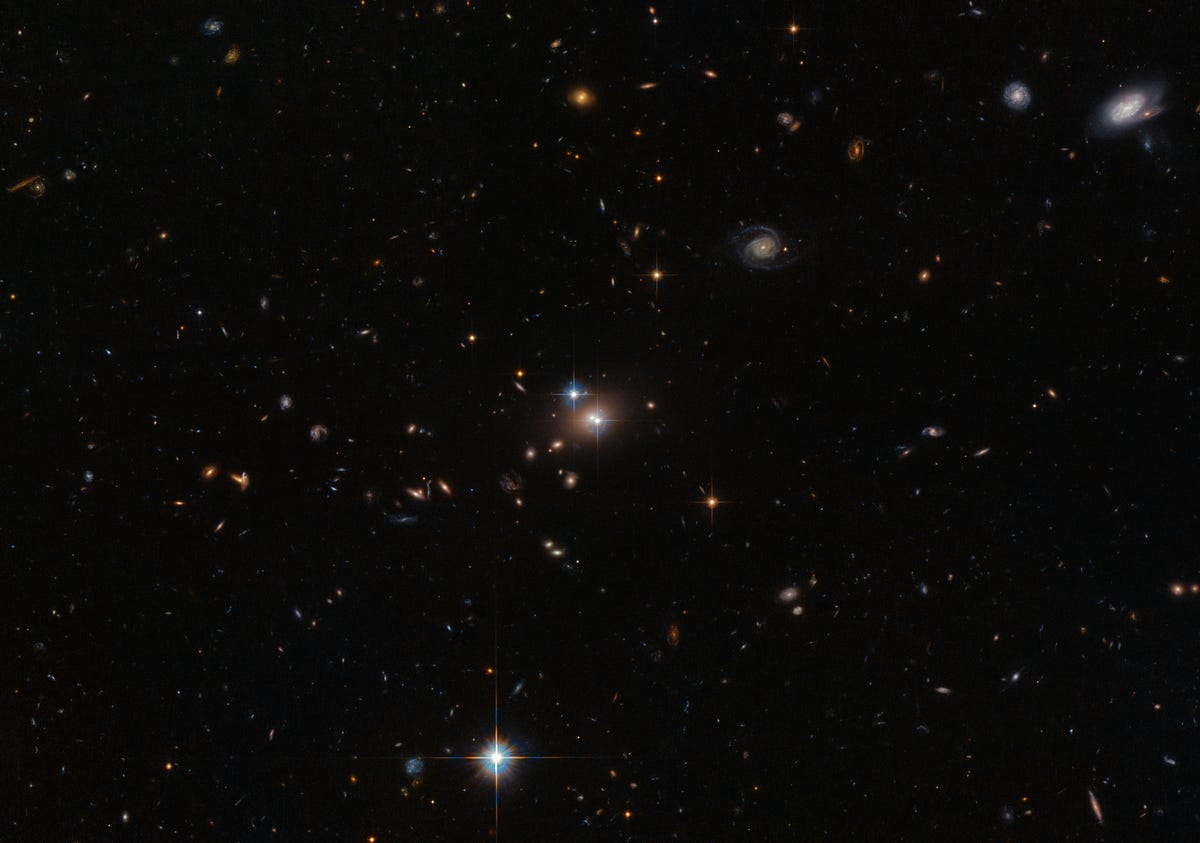
In addition to its failures on all scales larger than that of individual galaxies, it wasn’t a viable theory of gravity. It wasn’t relativistic, meaning it couldn’t explain things like the bending of starlight due to intervening mass, gravitational time dilation or redshift, the behavior of binary pulsars, or any other relativistic, gravitational phenomena verified to occur in agreement with Einstein’s predictions. The holy grail of MOND — and what many vocal proponents of dark matter demanded, including myself — was a relativistic version that could explain the rotation curves of galaxies along with all the other successes of our current theory of gravity.
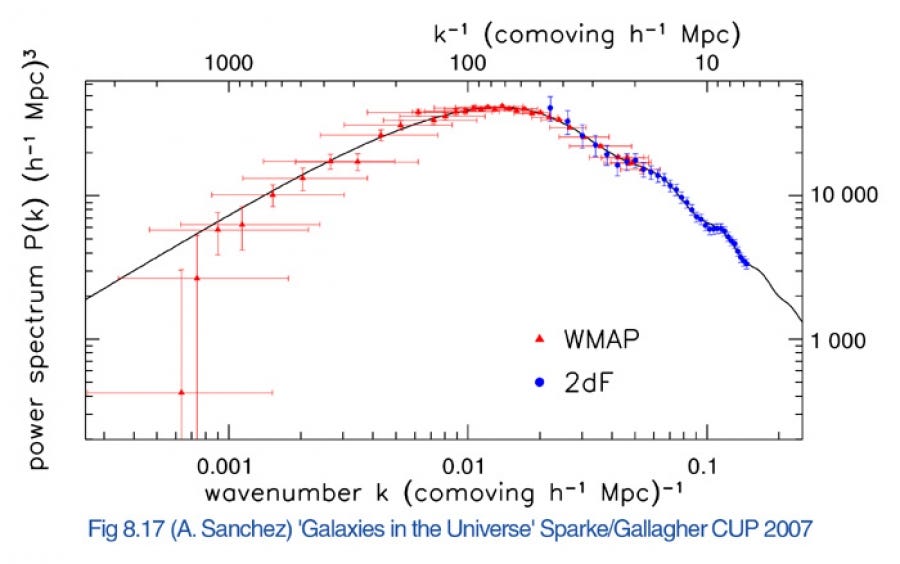
Meanwhile, as the years went on, dark matter started having a huge number of cosmological successes. As the large-scale structure of the Universe went from poorly-understood to well-understood, and as the matter power spectrum (above) and fluctuations in the cosmic microwave background (below) became precisely measured, dark matter was found to work wonderfully on the largest scales.
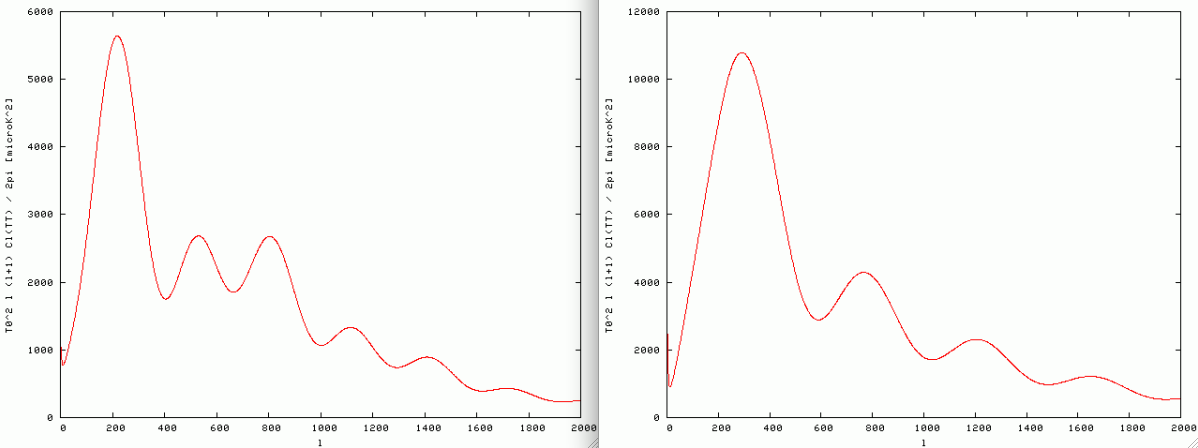
In other words, these new observations — just like those for Big Bang Nucleosynthesis — were consistent with a Universe that was composed of about five times as much dark (non-baryonic) matter as normal matter.
And then, in 2005, the supposed “smoking gun” was observed. We caught two galaxy clusters in the act of colliding, meaning that if dark matter was correct, we’d see the baryonic matter — the interstellar/intergalactic gas — colliding and heating up, while the dark matter, and hence the gravitational signal, should pass right through without slowing down. Below, you can see the X-ray data of the Bullet cluster in pink, with the gravitational lensing data overlaid in blue.
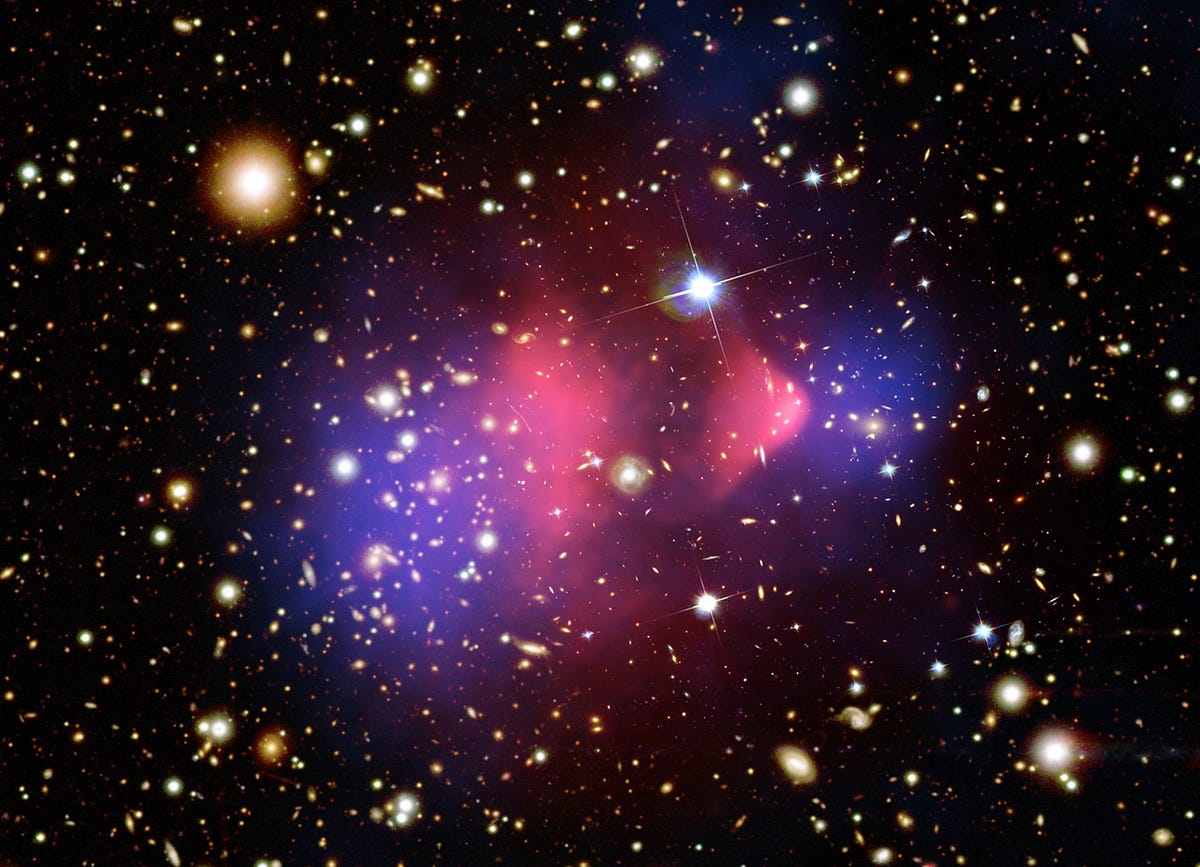
Lensing Map: NASA/STScI; ESO WFI; Magellan/U.Arizona/ D.Clowe et al.;
Optical: NASA/STScI; Magellan/U.Arizona/D.Clowe et al.
This was a huge victory for dark matter, and an equally huge challenge to all models of modified gravity. But small-scales still posed a problem for dark matter; it still isn’t as good at explaining the rotation of individual galaxies as MOND is. And thanks to TeVeS, a relativistic version of MOND formulated by Jacob Bekenstein, it looked like MOND would finally get a fair shot.
Gravitational lensing and some relativistic phenomena could be explained, and there was finally a clear-cut way to distinguish between the two: find an observational test where the predictions of TeVeS and the predictions of General Relativity differed from one another! Amazingly, such a setup already exists in nature.
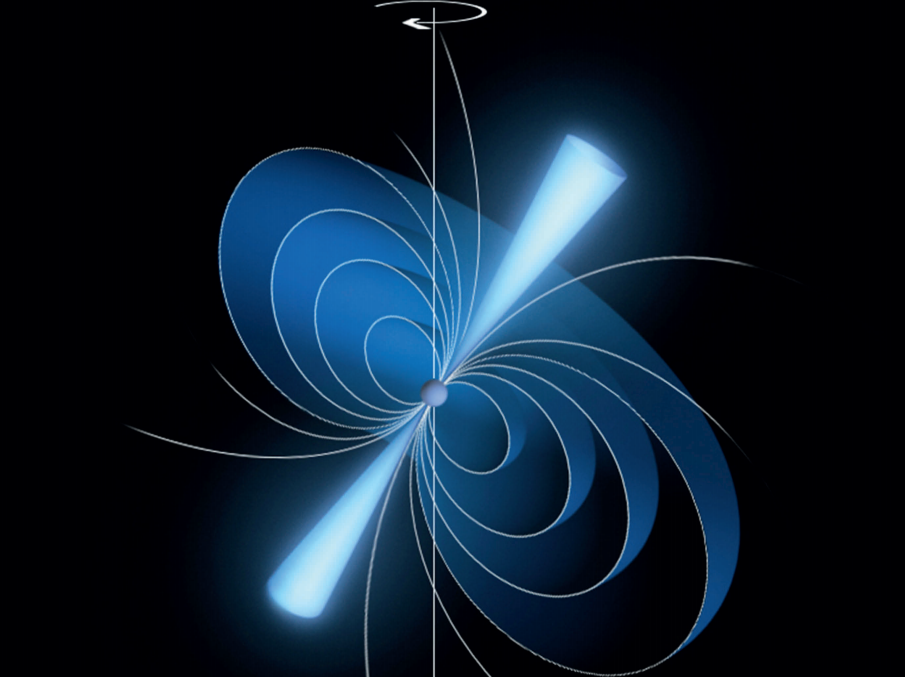
Spinning neutron stars — stellar remnants from ultramassive stars that have gone supernova and left a solar-mass atomic nucleus behind — are tiny things, only a few kilometers in diameter. Imagine that if you will: an object 300,000 times as massive as our planet, compressed down into a volume just one-hundred-millionth the size of our world! As you can imagine, gravitational fields near these guys get really intense, providing some of the most stringent strong-field tests of relativity ever.
Well, there are some instances where neutron stars have their axial “beams” pointed directly at us, so the “pulse” at us every time the neutron star completes an orbit, something that can happen up to 766 times a second for objects this small! (When this happens, the neutron stars are known as pulsars.) But in 2004, an even rarer system was discovered: a double pulsar!
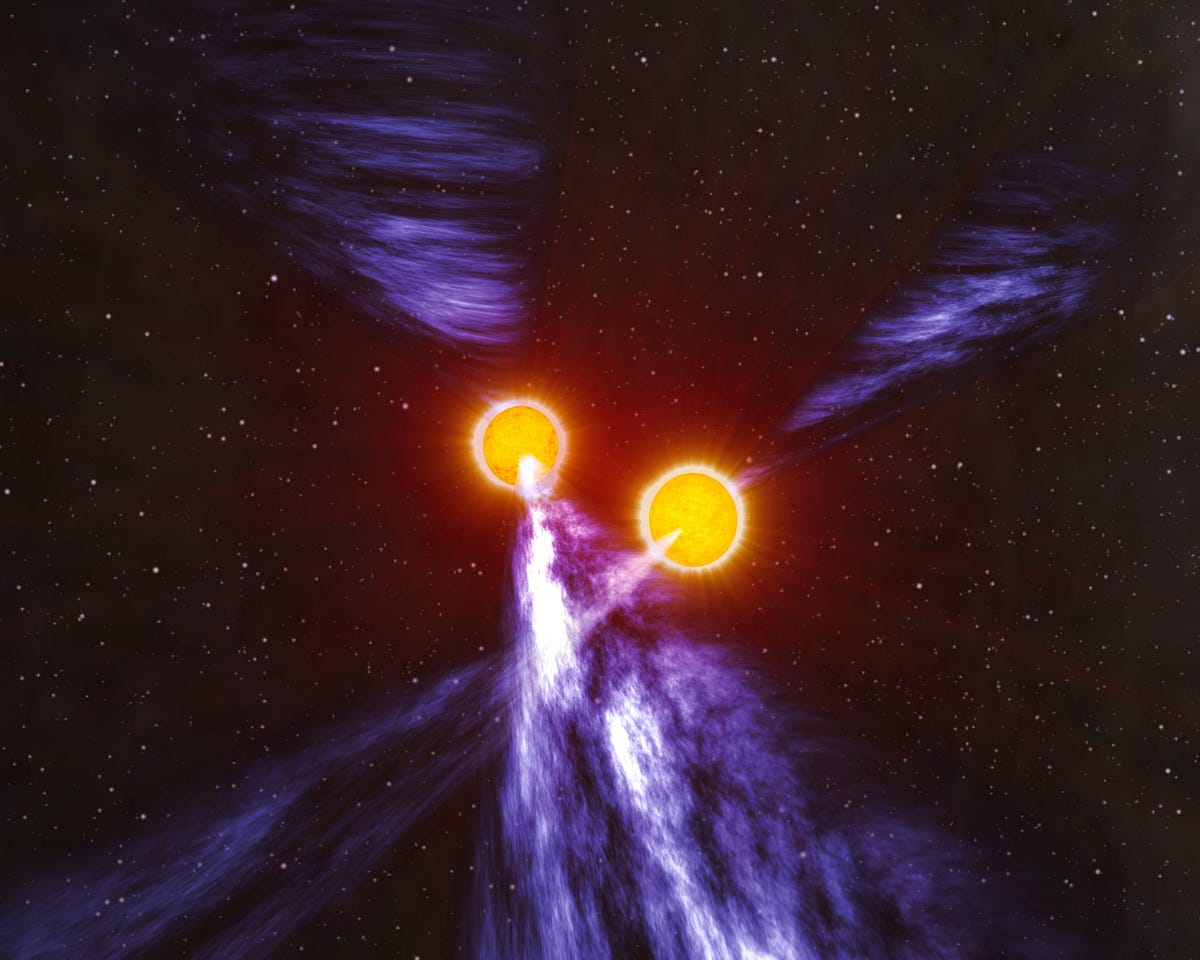
Over the past decade, this system has been observed in its very tight gravitational dance, and Einstein’s General theory of Relativity has been put to the test like never before. You see, as massive bodies orbit one another in very strong gravitational fields, they ought to emit a very specific amount of gravitational radiation. Although we don’t have the technology to measure these waves directly, we do have the ability to measure how the orbits are decaying due to this emission! Michael Kramer of the Max Planck Institute for Radio Astronomy was one of the scientists working on this, and here’s what he had to say about the orbits of this system (emphasis mine):
“We discovered that this causes the orbit to shrink by 7.12 millimeters a year, with an uncertainty of nine-thousandths of a millimeter.”
What do TeVeS and General Relativity have to say about this observation?
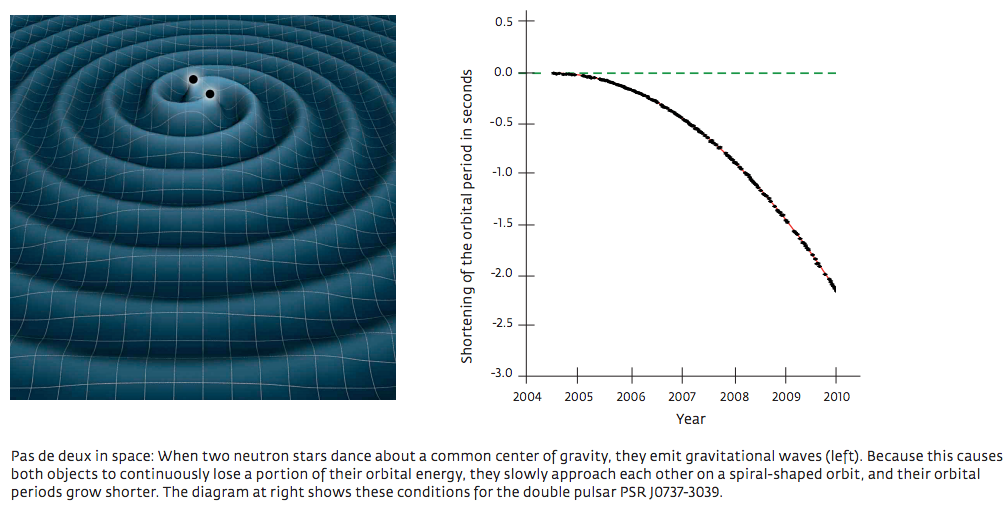
It agrees with Einstein’s relativity at the 99.95% level (with a 0.1% uncertainty), and — here’s the big one — rules out all physically viable incarnations of Bekenstein’s TeVeS. As scientist Norbert Wex said with unparalleled brevity,
“In our view, this refutes TeVeS.”
In fact, history’s most accurate simulation of structure formation (using General Relativity and dark matter) has just been released, and it agrees with all observations consistent to the limit of our technological capabilities. Watch the incredible video of Mark Vogelsberger and be amazed!
And with all that in mind, that’s why dark matter’s #1 competitor is no longer any competition at all.
Have a comment? Leave it at the Starts With A Bang forum on Scienceblogs!