What was it like when the Higgs gave mass to The Universe?
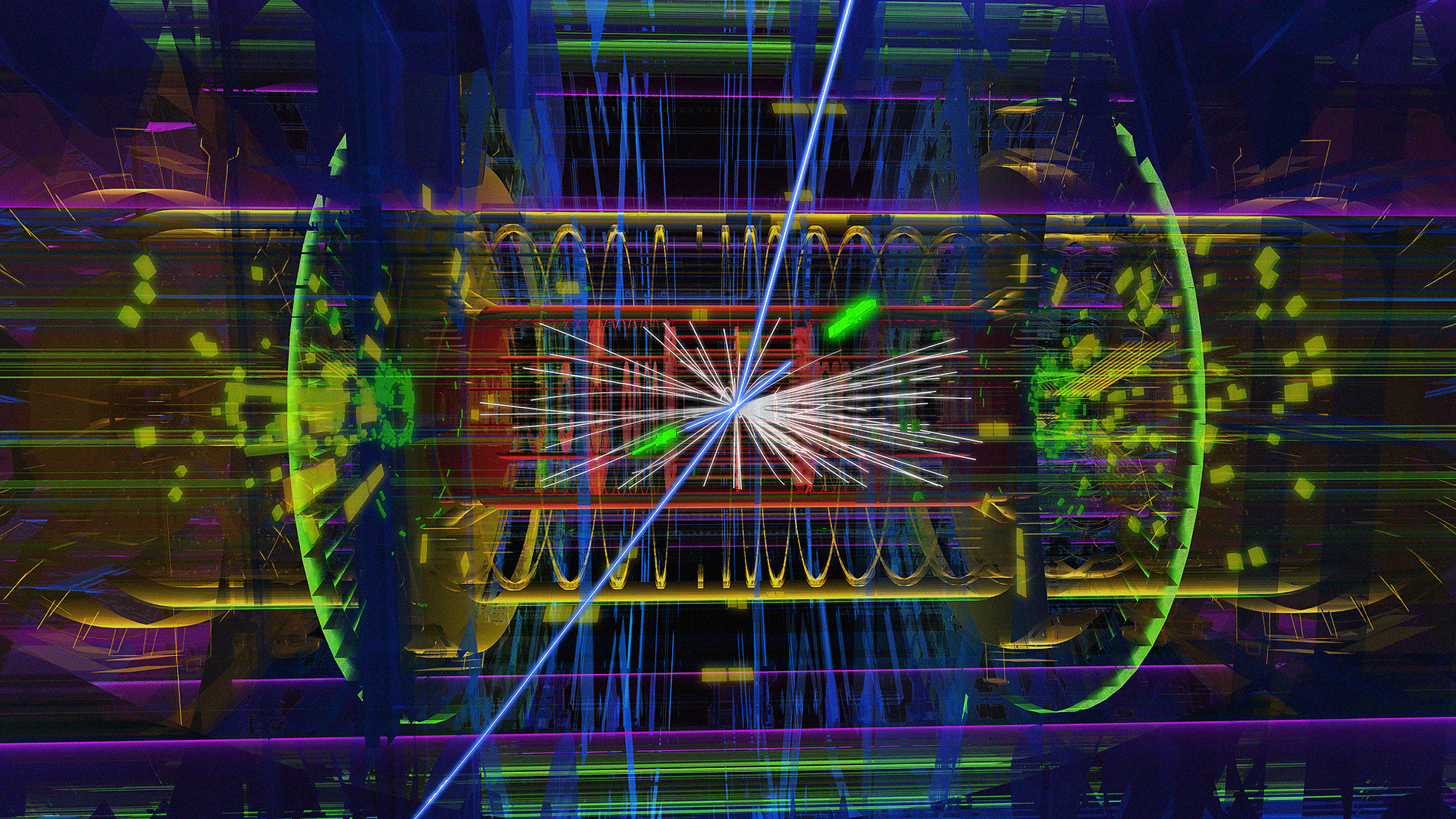
One moment, every particle in the Universe was massless. Then, they weren’t anymore. Here’s how it happened.
In the earliest stages of the hot Big Bang, the Universe was filled with all the particles, antiparticles, and quanta of radiation it had the energy to create. As the Universe expanded, it cooled: the stretching fabric of space also stretched the wavelengths of all the radiation within it to longer wavelengths, which equates to lower energies.
If there are any particles (and antiparticles) that exist at higher energies that are yet to be discovered, they were likely created in the hot Big Bang, so long as there was enough energy (E) available to create a massive (m) particle via Einstein’s E = mc². It’s possible that a slew of puzzles about our Universe, including the origin of the matter-antimatter asymmetry and the creation of dark matter, are solved by new physics at these early times. But the massive particles we know today are foreign to us. At these early stages, they have no mass.
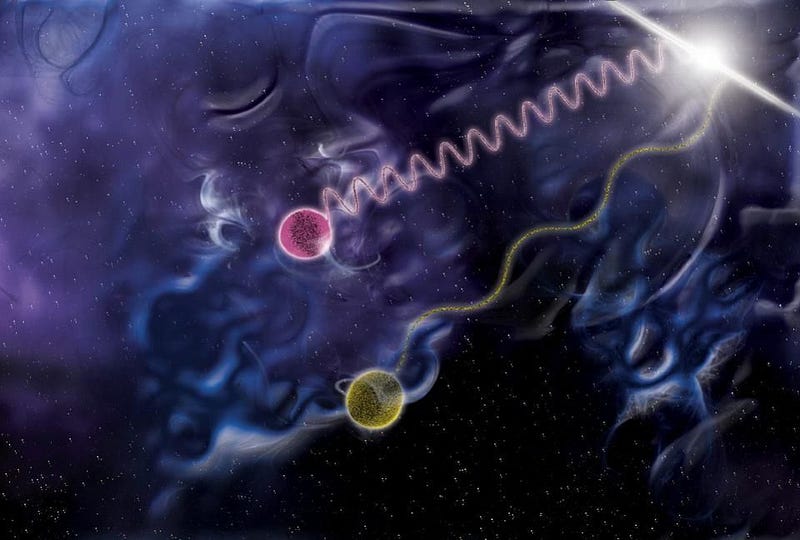
The particles and antiparticles of the Standard Model are easy to create, even as the Universe cools and the fractions-of-a-second ticked by. The Universe might start of at energies as large as 10¹⁵ or 10¹⁶ GeV; even by time it’s dropped to 1000 (10³) GeV, no Standard Model particle is threatened. At the energies achievable by the LHC, we can create the full suite of particle-antiparticle pairs that are known to physics.
But at this point, unlike today, they’re all massless. If they have no rest mass, they have no choice but to move at the speed of light. The reason particles are in this strange, bizarre state that’s so different from how they exist today? It’s because the fundamental symmetry that gives rise to the Higgs boson — the electroweak symmetry — has not yet broken in the Universe.

When we look at the Standard Model today, it’s arranged as follows:
- six quarks, each of which come in three colors, and their antiquark counterparts,
- three charged leptons (e, μ, τ) and three neutral ones (ν_e, ν_μ, ν_τ), and their antimatter counterparts,
- the eight massless gluons that mediate the strong force between the quarks,
- the three heavy, weak bosons (W+, W-, and Z_0) that mediate the weak nuclear force,
- and the photon (γ), the massless mediator of the electromagnetic force.
But there’s a symmetry that’s broken at today’s low-energy scale: the electroweak symmetry. This symmetry was restored in the early days of the Universe. And when it’s restored versus when it’s broken, it fundamentally changes the Standard Model picture.
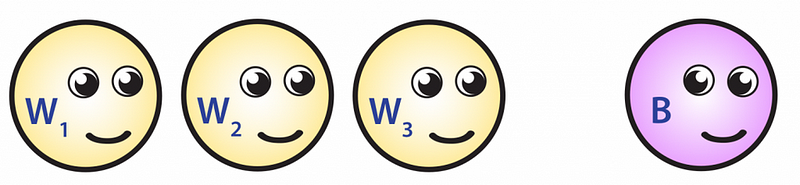
Instead of the weak and electromagnetic bosons (W+, W-, Z_0, γ), where the first three are very massive and the last is massless, we have four new bosons for the electroweak force (W_1, W_2, W_3, B), and all of them have no mass at all. The other particles are all the same, except for the fact that they, too, have no mass yet. This is what’s floating around in the early Universe, colliding, annihilating, and spontaneously being created, all in motion at the speed of light.
As the Universe expands and cools, all of this continues. So long as the energy of your Universe is above a certain value, you can think about the Higgs field as floating atop the liquid in a soda (or wine) bottle. As the level of the liquid drops, the Higgs field remains atop the liquid, and everything stays massless. This is what we call a restored-symmetry state.
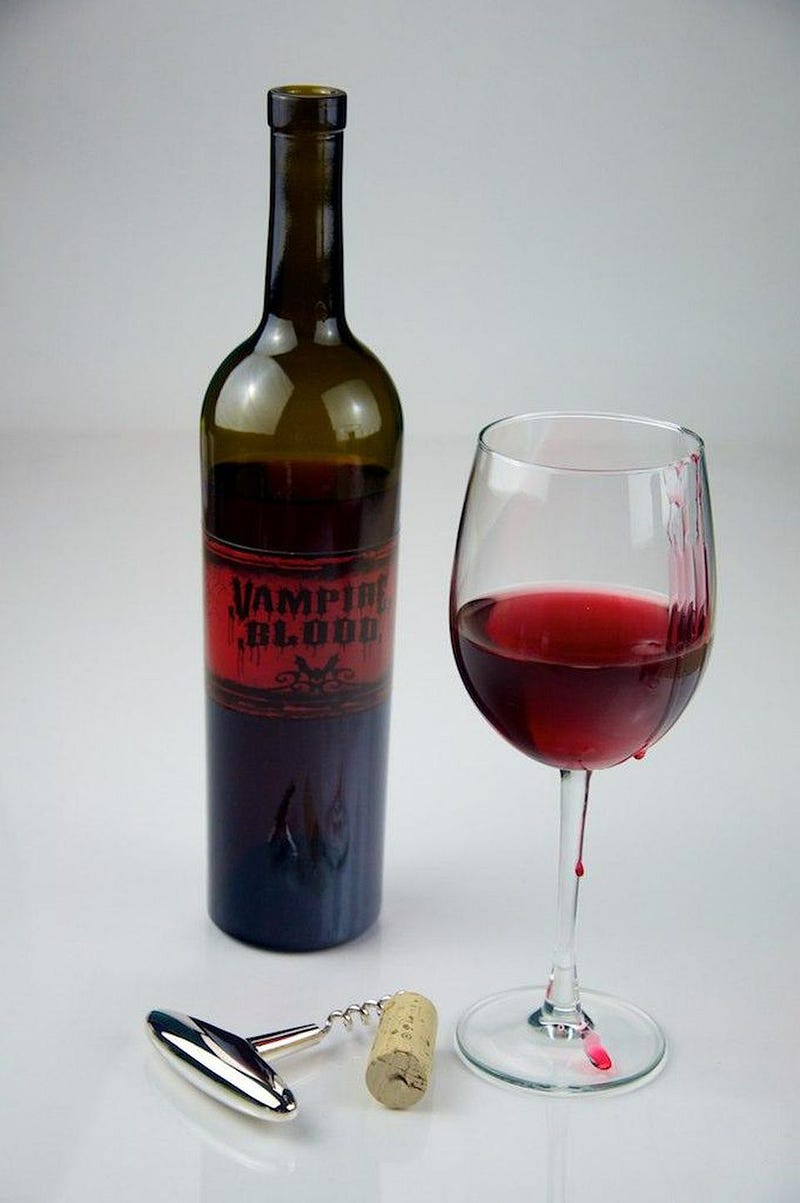
But below a certain liquid level, the bottom of the container starts to show itself. And the field can no longer remain in the center; more generally, it can’t take on simply any old value. It has to go to where the liquid level is, and that means down into the divot(s) at the bottom of the bottle. This is what we call a broken-symmetry state.
When this symmetry breaks, the Higgs field settles into the bottom, lowest-energy, equilibrium state. But that energy state isn’t quite zero: it has a finite, non-zero value known as its vacuum expectation value. Whereas the restored-symmetry state yielded only massless particles, the broken symmetry state changes everything.

Once the symmetry breaks, the Higgs field has four mass-containing consequences: two are charged (one positive and one negative) and two are neutral. Then, the following things all happen at once:
- The W_1 and W_2 particles “eat” the charged, broken-symmetry consequences of the Higgs, becoming the W+ and W- particles.
- The W_3 and B particles mix together, with one combination eating the uncharged broken-symmetry consequence of the Higgs, becoming the Z_0, and with the other combination eating nothing, to remain the massless photon (γ).
- The last neutral broken-symmetry consequence of the Higgs gains mass, and becomes the Higgs boson.
- At last, the Higgs boson couples to all the other particles of the Standard Model, giving mass to the Universe.
This is the origin of mass in the Universe.
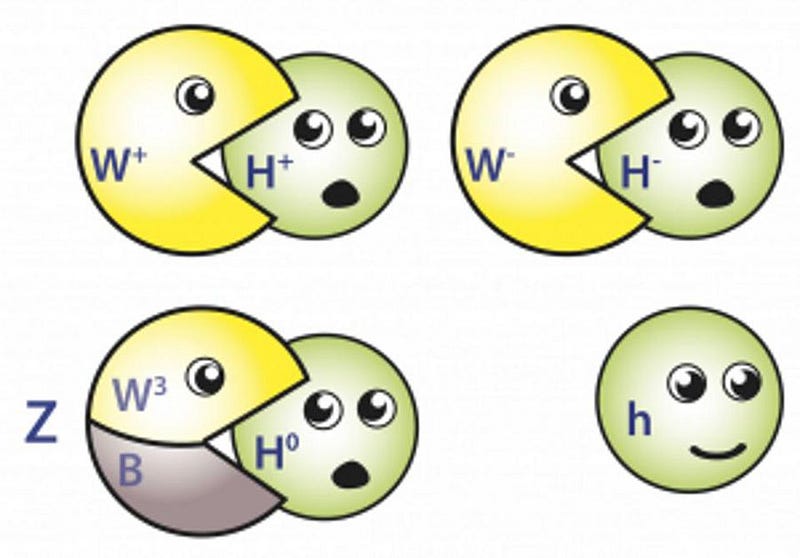
This whole process is called spontaneous symmetry breaking. And for the quarks and leptons in the standard model, when this Higgs symmetry is broken, every particle gets a mass due to two things:
- The expectation value of the Higgs field, and
- A coupling constant.
And this is kind of the problem. The expectation value of the Higgs field is the same for all of these particles, and not too difficult to determine. But that coupling constant? Not only is it different for every particle, but — in the standard model — it’s arbitrary.
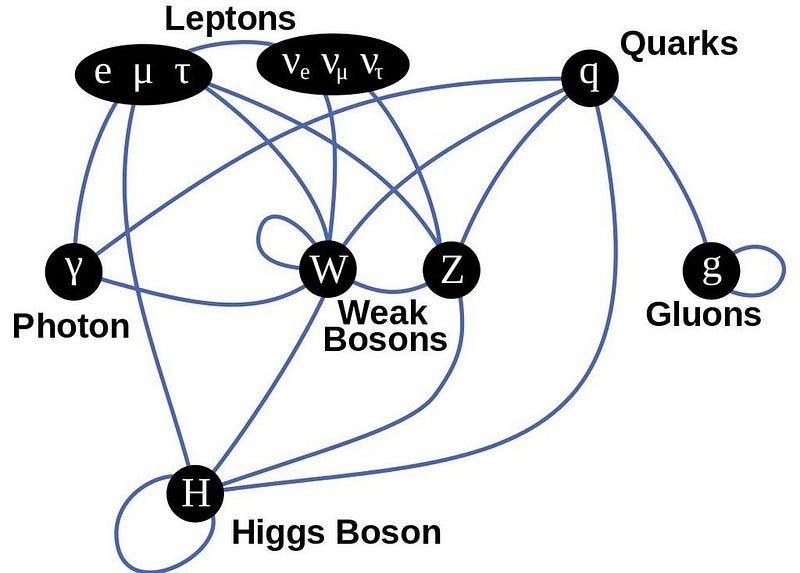
We know that the particles have mass; we know how they get mass; we’ve discovered the particles responsible for mass. But we still have no idea why the particles have the values of the masses they do. We have no idea why the coupling constants have the couplings that they do. The Higgs boson is real; the gauge bosons are real; the quarks and leptons are real. We can create, detect, and measure their properties exquisitely. Yet, when it comes to understanding why they have the values that they do, that’s a puzzle we cannot yet solve. We do not have the answer.
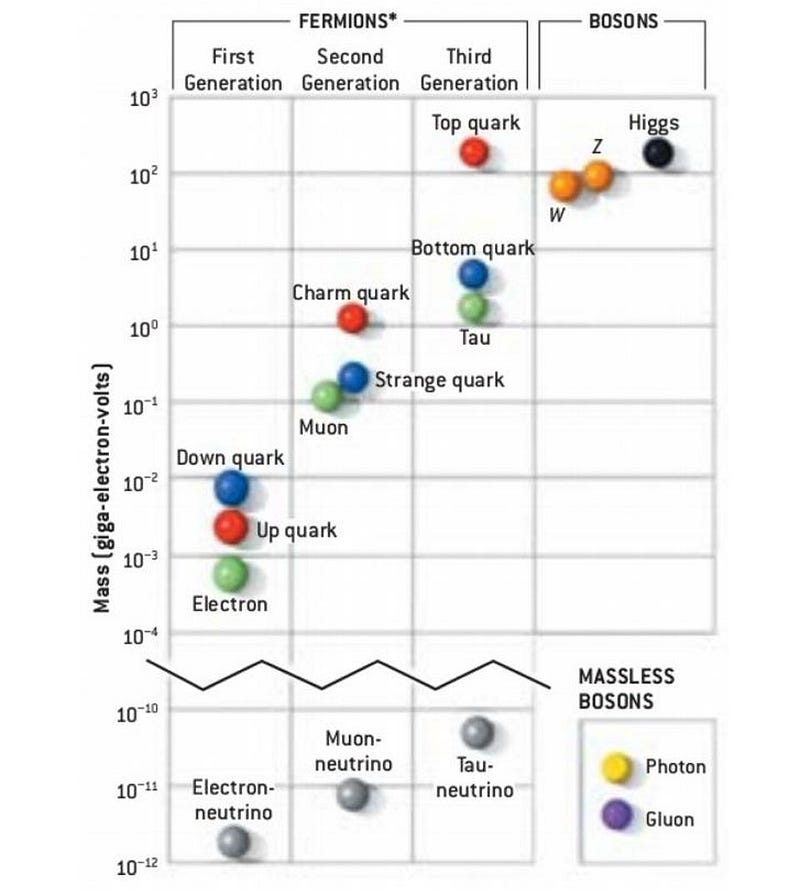
Before the breaking of the electroweak symmetry, everything that is known to exist in the Universe today is massless, and moves at the speed of light. Once the Higgs symmetry breaks, it gives mass to the quarks and leptons of the Universe, the W and Z bosons, and the Higgs boson itself. Suddenly, with huge mass differences between light particles and heavy ones, the heavy ones spontaneously decay into the lighter ones on very short timescales, especially when the energy (E) of the Universe drops below the mass equivalent (m) needed to create these unstable particles via E = mc².
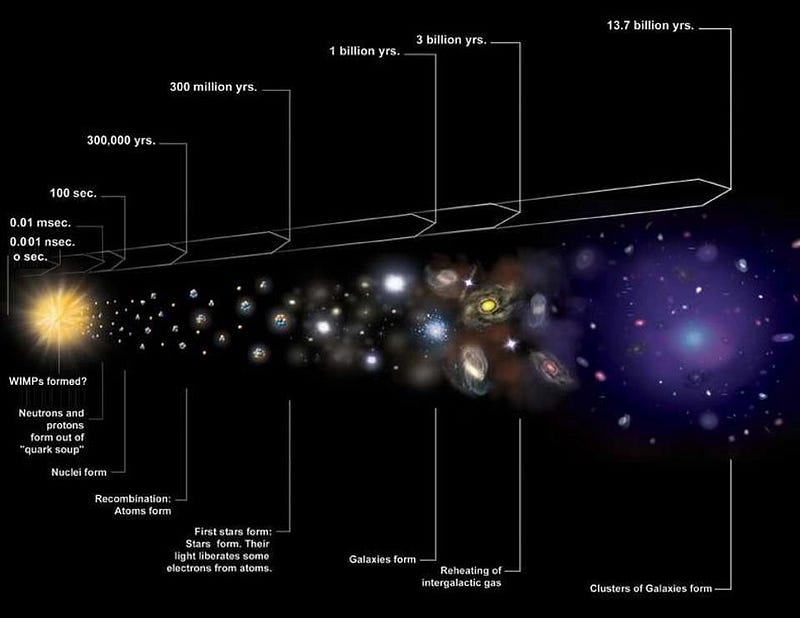
Without this critical gauge symmetry associated with electroweak symmetry breaking, existence wouldn’t be possible, as we do not have stable, bound states made purely of massless particles. But with fundamental masses to the quarks and charged leptons, the Universe can now do something it’s never done before. It can cool and create bound states like protons and neutrons. It can cool further and create atomic nuclei and, eventually, neutral atoms. And when enough time goes by, it can give rise to stars, galaxies, planets, and human beings. Without the Higgs to give mass to the Universe, none of this would be possible. The Higgs, despite the fact that it took 50 years to discover, has been making the Universe possible for 13.8 billion years.
Further reading on what the Universe was like when:
- What was it like when the Universe was inflating?
- What was it like when the Big Bang first began?
- What was it like when the Universe was at its hottest?
- What was it like when the Universe first created more matter than antimatter?
Ethan Siegel is the author of Beyond the Galaxy and Treknology. You can pre-order his third book, currently in development: the Encyclopaedia Cosmologica.