What was it like when the last antimatter disappeared?
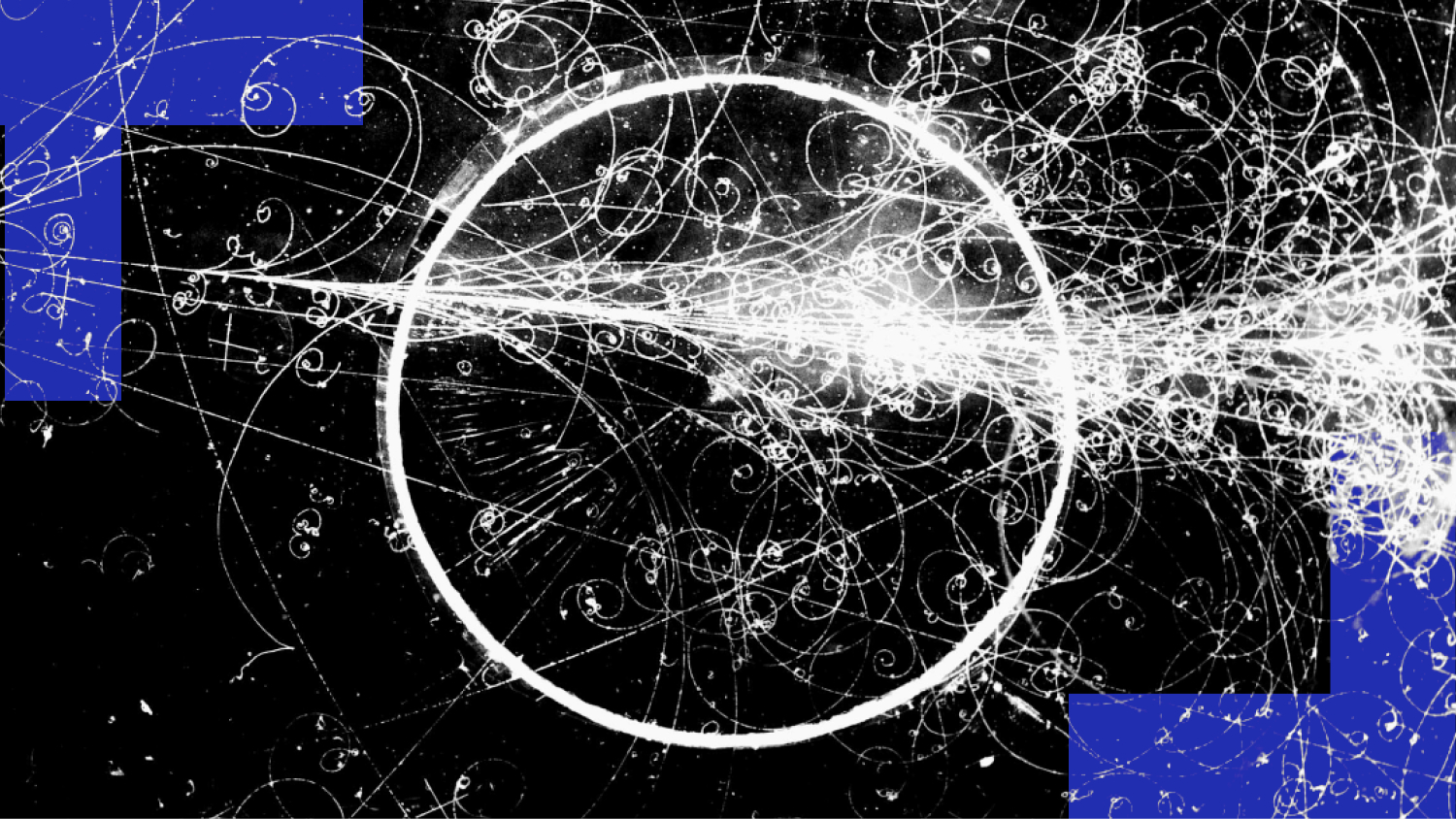
- In the earliest stages of the hot Big Bang, every possible particle and antiparticle that could have been created came into existence, in enormous numbers and in rapid fashion.
- As the Universe expanded and cooled, however, unstable particles and antiparticles decayed and annihilated away while becoming more difficult to create, eventually leaving a slight excess of matter.
- But different species of antimatter hung around for different amounts of time, with large numbers of positrons, in particular, playing a big role in the early Universe. Today, only antineutrinos remain for antimatter.
Things happen fast in the earliest stages of the Universe. In the first 25 microseconds after the start of the hot Big Bang, a number of incredible events have already occurred. The Universe created all the particles and antiparticles — known (as part of the Standard Model) and unknown (including whatever makes up dark matter) — it was ever capable of creating, reaching the highest temperatures it ever attained. Through a still-undetermined process, it created an excess of matter over antimatter: just at the 1-part-in-a-billion level. The electroweak symmetry broke, allowing the Higgs to give mass to the Universe. The heavy, unstable particles decayed away, and the quarks and gluons bound together to form protons and neutrons.
But that only gets us so far. At these early stages, there may be protons and neutrons in the Universe, as well as a high-energy bath of photons and neutrinos-and-antineutrinos, but we’re still a long way from the Universe as we recognize it today. In order to get there, a number of other things must occur. And the first of those, once we have protons and neutrons, is to get rid of the last of our antimatter, which is still incredibly abundant.
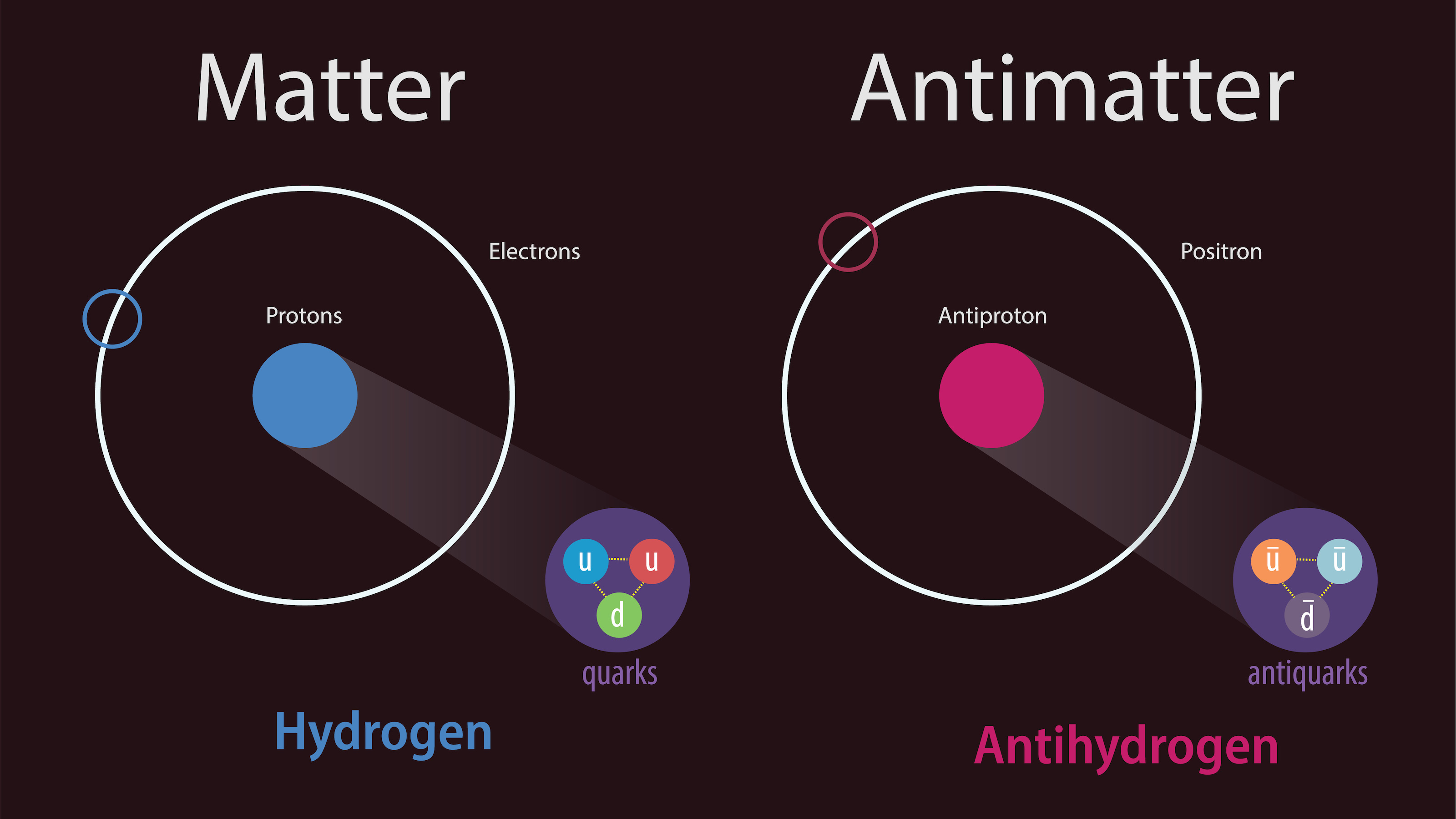
You can always make antimatter in the Universe, so long as you have the energy for it. Einstein’s most famous equation, E = mc², works two ways, and it works equally well for both applications.
- It can create energy from pure matter (or antimatter), converting mass (m) into energy (E) by reducing the amount of mass present, such as by annihilating equal parts matter with antimatter.
- Or it can create new matter from pure energy, so long as it also makes an equivalent amount of the antimatter counterparts for each matter particle it creates.
These annihilation-and-creation processes, so long as there’s enough energy for creation to proceed smoothly, balance out in the early Universe.
Early on, when the Universe was very hot, this process easily allowed us to create all of the particles and antiparticles contained within the Standard Model, as even the most massive particle (or antiparticle) known — the top quark — can be created quite easily: so long as there’s more than ~175 GeV of energy (the rest-mass energy of the top quark and antiquark) available for new particle (or antiparticle) creation with each typical collision that occurs.

So that’s how the hot Big Bang starts off: with this hot particle-antiparticle soup made up of all allowable species. In the earliest stages, it’s the heaviest particle-antiparticle pairs that disappear first. It takes the most energy to create the most massive particles and antiparticles, so as the Universe cools, it gets progressively less and less likely that the quanta of energy that interact can spontaneously create new particle/antiparticle pairs.
By the time the Higgs gives mass to the Universe, this primordial particle/antiparticle soup is too low in energy to create top quarks or W-and-Z bosons. Shortly thereafter, it becomes no longer possible to spontaneously create:
- bottom quarks,
- tau leptons,
- charm quarks,
- strange quarks,
- or even muons (in that order).
Right around that same time that muons and antimuons annihilate and decay away, quarks-and-gluons bind together into neutrons and protons, while antiquarks bind together into antineutrons and antiprotons.
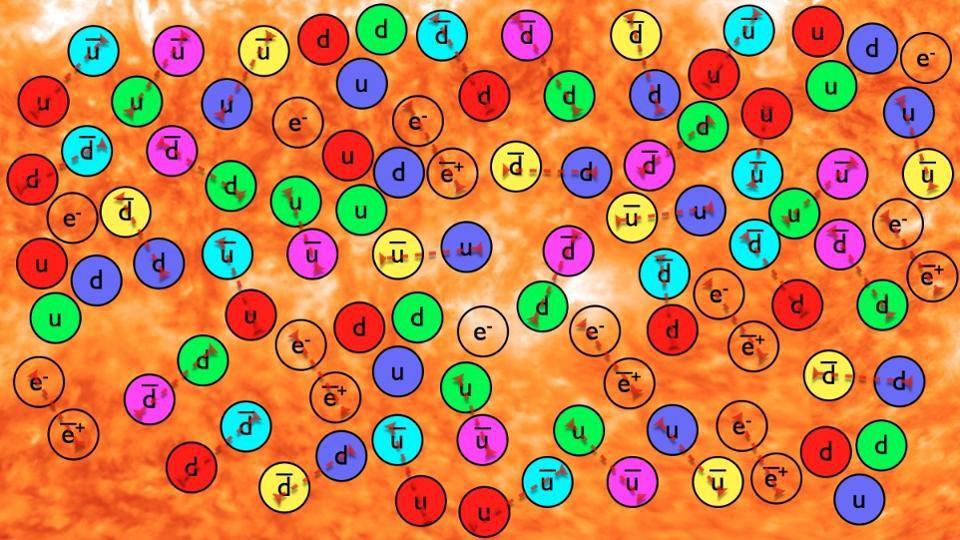
While there was plenty of energy available to create free up/anti-up and down/anti-down quarks, the onset of what we call “confinement” (or the hadron era) in the Universe means that such interactions are no longer possible; you must create whole protons/antiprotons or neutrons/antineutrons, which are much more massive that the quarks that compose them. The energy available in the Universe is far too low for that to occur, so all the antimatter, in the form of antiprotons and antineutrons, annihilates away with as much matter as it can find.
However, since there is somewhere around 1 extra proton (or neutron) for every 1.4 billion proton/antiproton pairs, we’re left over with a small excess of protons and neutrons.
All of the proton/antiproton and neutron/antineutron annihilations give rise to photons — the purest form of raw energy — along with all the prior annihilations that gave rise to photons as well. Photon-photon interactions are still going strong at this early, energetic stage, and they can spontaneously produce both neutrino-antineutrino pairs and electron-positron pairs. Even after we make protons and neutrons, and even after all the antiprotons and antineutrons have disappeared, the Universe is still rife with antimatter: in the form of antineutrinos and positrons.

It’s important to remember, even at this relatively late stage in the game (tens of microseconds after the start of the hot Big Bang), how hot and dense things still truly are. The Universe has had just a fraction of a second go by since the Big Bang, and particles are packed tighter everywhere than they are, today, within the center of our Sun. The ambient temperatures would have to be measured in the trillions of degrees: more than 100,000 times as great as in the Sun’s core. And perhaps most importantly, there are a slew of interactions constantly occurring that can transform one type of particle into another.
Today, we’re used to the weak nuclear interactions occurring spontaneously in one context alone: that of radioactive decay. Higher-massed particles, like a free neutron or a heavy atomic nucleus, emit daughter particles that are less massive, giving off some energy in accord with that same equation Einstein put forth: E = mc². But at these stages of the Big Bang, even after the electroweak symmetry breaks, the weak interactions continue to play a more important role than simply being responsible for radioactive decays for some time.

In the hot, dense, early Universe, there’s a second role for the weak interaction to play, enabling protons and neutrons to convert into one another. So long as the Universe is energetic enough, here are four extreme reactions that occur spontaneously:
- p + e– → n + νe,
- n + e+ → p +
νe, - n + νe → p + e–,
- p +
νe → n + e+.
In these equations, p is for proton, n is for neutron, e– is for electron, e+ is for positron (antielectron), while νe is an electron-neutrino and νe is an electron-antineutrino.
You’ll also notice that, when it comes to these four equations, equations #1 and #3 are simply the inverse of one another, while equations #2 and #4 are also the inverse of one another. This is an indication to us that these reactions can proceed either forward (e.g., where protons and electrons interact, resulting in a neutron and neutrino) or backward (e.g., where neutrons and neutrinos interact, resulting in a proton and electron), so long as the weak interactions and the amount of available energy both allow these reactions to proceed.
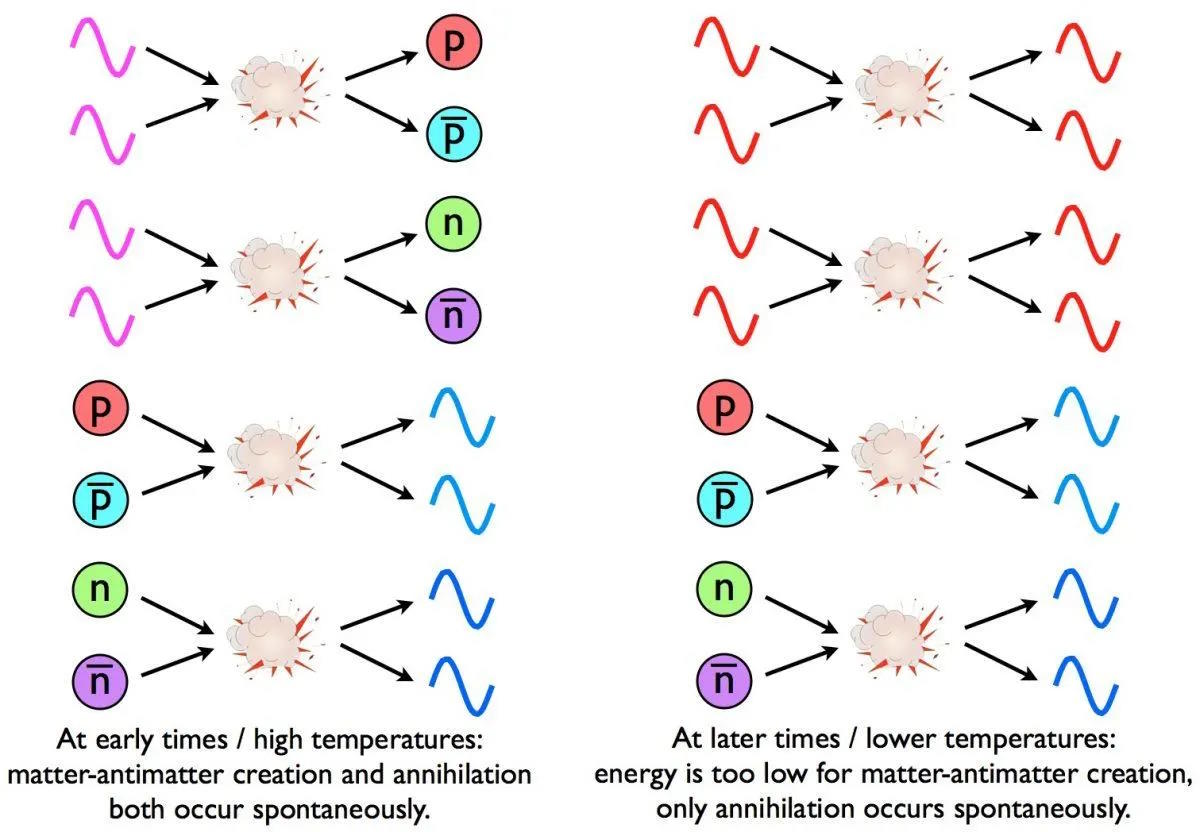
So long as temperatures and densities are high enough, all of these reactions occur spontaneously and at equal rates. Under these conditions:
- the weak interactions are still important,
- there’s a strong enough coupling between protons/neutrons and electrons/positrons/neutrinos/antineutrinos,
- there’s enough matter and antimatter for these reactions to occur frequently,
- and there’s enough energy to create higher-massed neutrons out of lower-massed protons.
While protons/neutrons form and the excess antiprotons/antineutrons are all gone only a few tens of microseconds after the start of the hot Big Bang, the aforementioned conditions are all met for about the first full second after the Big Bang. During this time, everything is in equilibrium, and the Universe interconverts protons-and-neutrons at will, giving us about a 50/50 split between protons and neutrons while this is the case. Each time you convert a proton into a neutron, it’s just as easy to convert a neutron into a proton, and these reactions happen at about the same overall net rate.
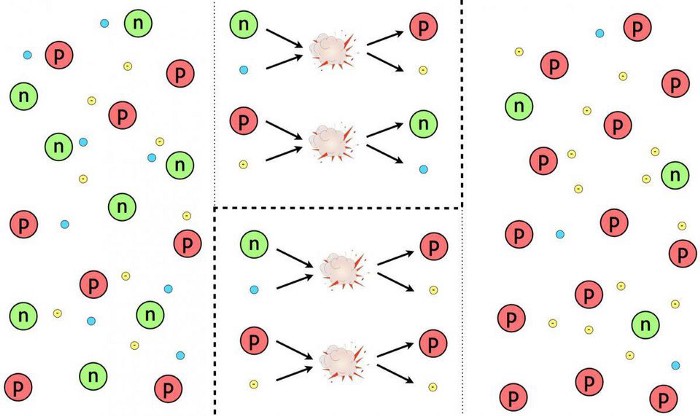
But this doesn’t remain the case forever, or even for that long. As the energy inherent to each particle drops, it becomes just a little bit more energetically favorable to produce a proton than a neutron from these interactions. The neutron, remember, is just a little bit more massive than the proton, and is even a little more massive than a proton and an electron combined. As a result, when the temperature of the Universe drops to a value that corresponds to that energy difference, the proton population begins to slightly dominate the neutron population. This occurs right around the time the Universe reaches an age of one second after the Big Bang.
But then, at that moment, two additional things happen in rapid succession, forever altering the course of the Universe.
The first is that the weak interactions freeze out, meaning that the proton-neutron interconversion interactions stop occurring. These interconversions required neutrinos to interact with protons and neutrons at a certain frequency, which they could so long as the Universe was hot and dense enough. When the Universe gets cold and sparse enough, the neutrinos (and antineutrinos) no longer interact, meaning that the neutrinos and antineutrinos we’ve made at this point simply ignore everything else in the Universe. They should still be around at present, with a kinetic energy that corresponds to a temperature (assuming neutrinos are massless, which they aren’t quite) of just 1.95 K above absolute zero.

On the other hand, the Universe is still energetic enough that when two photons collide, they can still spontaneously produce electron-positron pairs, and where electron-positron pairs annihilate into two photons. This continues for just a tiny bit longer: until the Universe is about three seconds old (as opposed to the one second freeze out for neutrinos). This “second additional thing,” occurring just slightly after the weak interactions freeze out, means that all the matter-antimatter energy that was tied up in electrons and positrons goes exclusively into photons, and not into species of neutrinos-and-antineutrinos, when they annihilate.
This annihilation, of electrons and positrons into photons, represents the Universe losing the last of its antimatter. After this event, only antineutrinos, which already stopped interacting with the other particles in the Universe something like ~2 seconds ago, remain and persist up through and including the present day.
This has a big implication for the temperature of the leftover photon background — known today as the Cosmic Microwave Background — that it should be exactly (11/4)1/3 times hotter than the neutrino background: a temperature of 2.73 K instead of 1.95 K. Believe it or not, we’ve already detected both of these backgrounds and measured their temperature (for photons) or temperature-equivalent (for neutrinos/antineutrinos), and they match with these explicit predictions from the Big Bang perfectly.

The Cosmic Microwave Background, although it was first detected in 1964, required a very high precision set of measurements to determine its temperature. Although many efforts and improvements occurred throughout the 1960s, 70s, and 80s, the CMB’s temperature was only first measured to this incredible precision back in 1992, with the first data release of NASA’s COBE satellite. (That data is shown above.)
However, the neutrino background imprints itself onto the CMB and in the large-scale structure of the Universe only in a very subtle way, and the evidence for that neutrino background and its properties wasn’t first detected until 2015. When it was finally discovered, the scientists who did the work found a phase shift in the Cosmic Microwave Background’s fluctuations that enabled them to determine, if neutrinos were massless today, how much energy they’d have at this early time.
Their results? The Cosmic Neutrino Background had an equivalent temperature of 1.96 ± 0.02 K, in perfect agreement with the Big Bang’s predictions. Later work, in 2019, found additional evidence for the Cosmic Neutrino Background imprinted in the Universe’s large-scale structure, but with less precision than the CMB method.
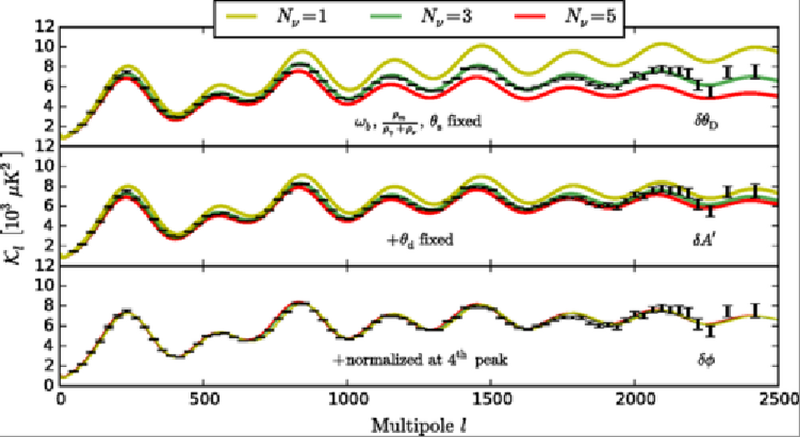
You might wonder why it’s worth belaboring such a tiny detail in the early Universe, and the answer is profound. Because of the brief amount of time that:
- the weak interactions were important (during the first ~1 second after the hot Big Bang),
- and antimatter also persisted (during the first ~3 seconds after the hot Big Bang),
the Universe is not evenly split, 50/50, between protons and neutrons any longer. Rather, the split has shifted substantially: to be more like 85/15, in favor of protons over neutrons. With the neutrinos and antineutrinos completely decoupled from all the other particles in the Universe, they simply move through space freely, at speeds indistinguishable (but slightly lower than) the speed of light. Meanwhile, the positrons (i.e., antielectrons) are all gone, and so are most of the electrons.
When the dust clears, what’s left over are exactly as many electrons as there are protons, keeping the Universe electrically neutral. There are over a billion photons for every proton or neutron, with another background of about 70% as many neutrinos-and-antineutrinos as photons. The Universe is still hot and dense, but it’s cooled tremendously in just the first 3 seconds. Now that all the antimatter is gone, the raw ingredients to begin building the Universe as we know it are finally in place.