What was it like when protons and neutrons formed?
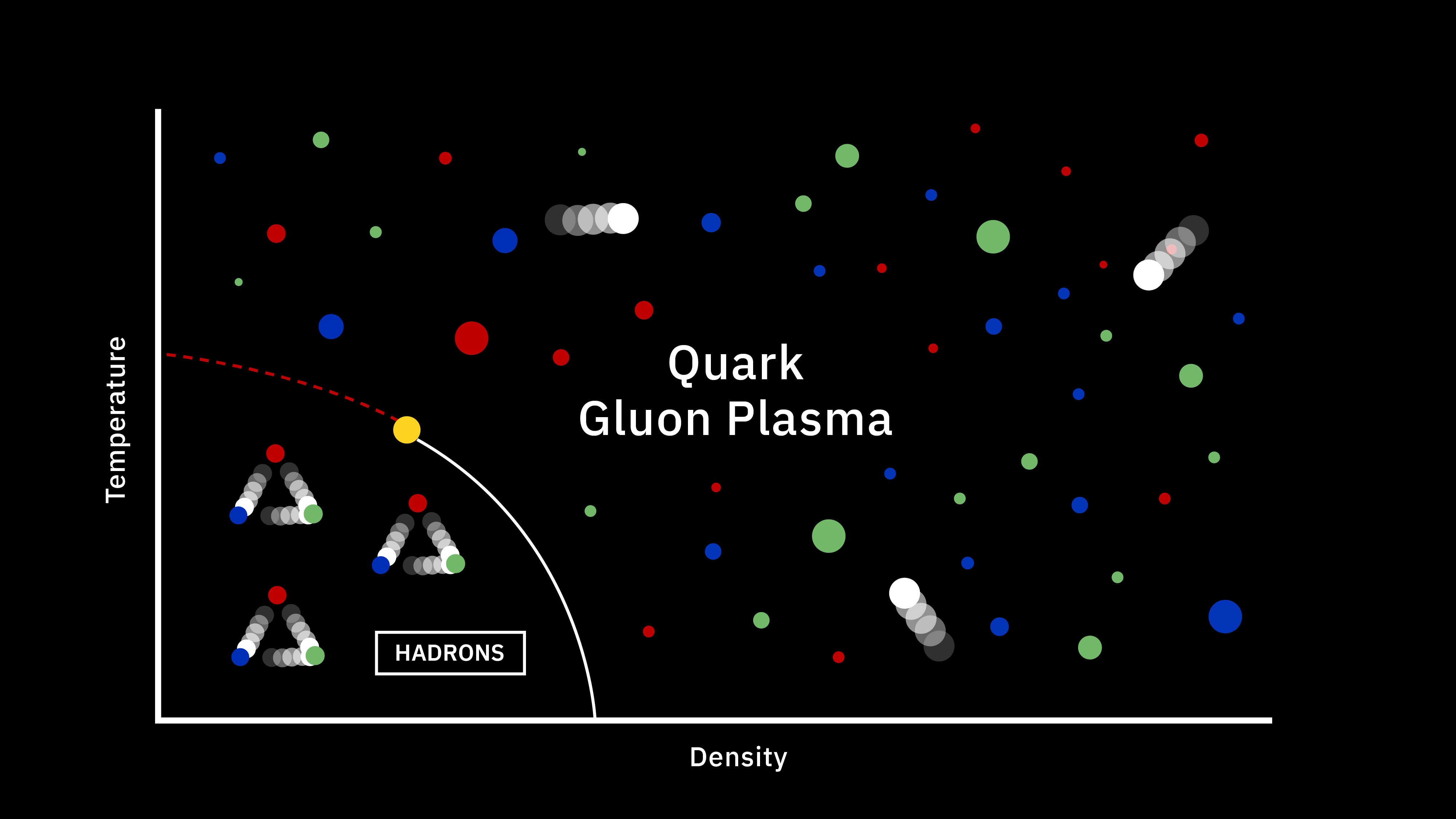
- At very high temperatures and densities, including the conditions found in the early stages of the hot Big Bang, there was no such thing as a proton or neutron, only a quark-gluon plasma.
- Through heavy ion collisions within powerful particle accelerators, we’ve managed to recreate those quark-gluon plasma conditions in the lab, with fascinating consequences.
- As a result, we now understand what it was like when protons and neutrons first formed better than ever. Here’s the cosmic story of what it was like when that first occurred.
The story of our cosmic history is one of an expanding and cooling Universe. As we progressed from a hot, dense, uniform state to a cold, sparse, clumpy one, a number of momentous events happened throughout our cosmic history. At the moment of the hot Big Bang, the Universe was filled with all sorts of ultra-high energy particles, antiparticles, and quanta of radiation, moving at or close to the speed of light.
On the other hand, today, we have a Universe filled with stars, galaxies, gas, dust, and many other phenomena that are too low in energy to have existed in the early Universe. Once things cooled enough so that the Higgs gave mass to the Universe, you might think that protons and neutrons would have immediately formed thereafter.
But under the hot, dense conditions that were present in the young, post-Big Bang Universe, they couldn’t exist right away. It took time, and the right set of conditions, before even something as fundamental to our Universe as a proton or neutron could stably form for the first time. Here’s the story of how those essential building blocks of atoms first came to be.
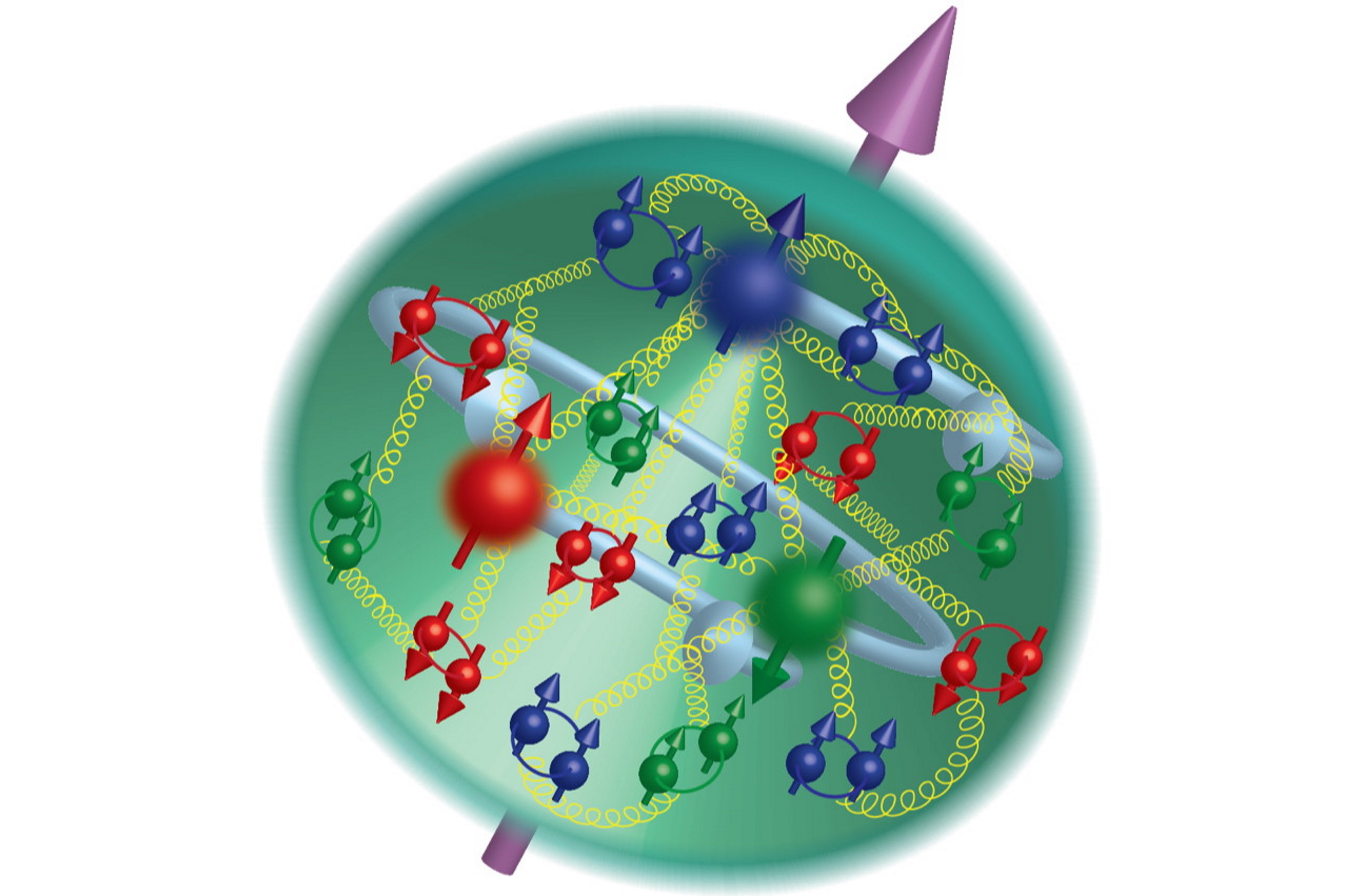
In the hot, dense environment of the early Universe, but after the fundamental particles have obtained a rest mass, the Universe was teeming not only with high-energy radiation, but also with several species of fast-moving, relativistic particles. In fact, the Universe possesses every particle-antiparticle combination that’s energetically possible in great numbers, continuously popping in-and-out of existence as particle-antiparticle pairs emerge from the collisions of other quanta. The particles that exist at this time include:
- quarks and antiquarks,
- leptons and antileptons,
- neutrinos and antineutrinos,
- as well as the gauge bosons.
So long as there’s enough energy (E) to create these particles of given masses (m) via Einstein’s E = mc2, they’re going to continue to exist in great abundance, in whatever equilibrium conditions cause “creation” and “annihilation” events to happen at the same rate. In terms of time after the start of the hot Big Bang, particles get their rest mass just 100 picoseconds (10-10 s) after the hot Big Bang begins: about the time it takes for light to travel a whopping three centimeters. At this early stage, there are no protons or neutrons in existence just yet.
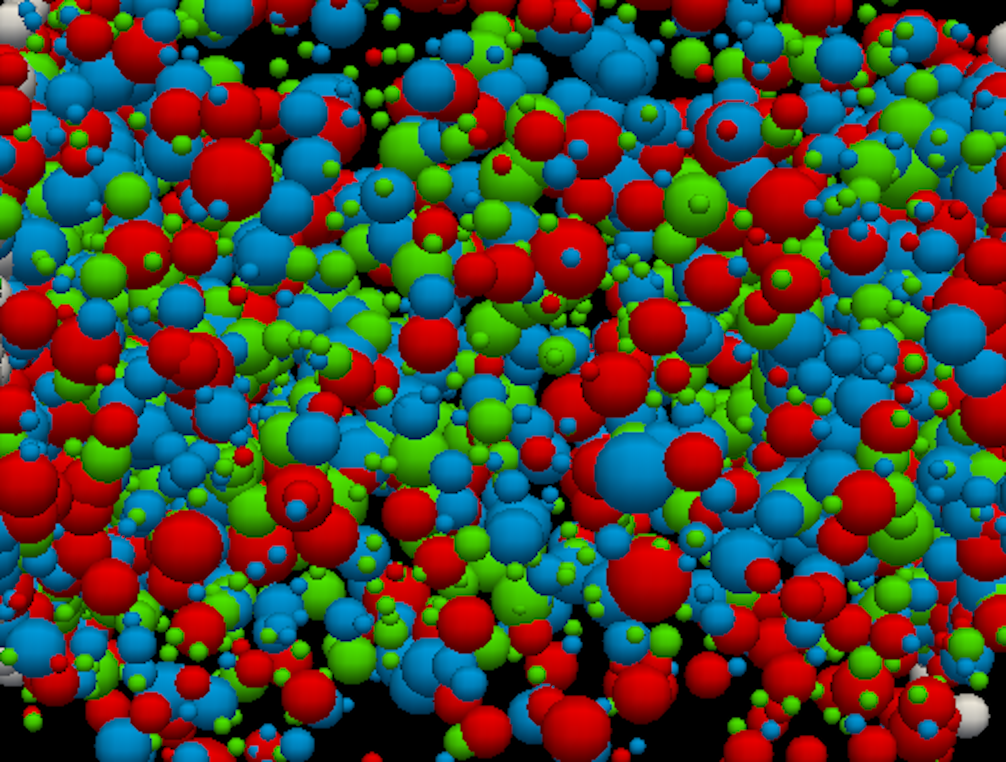
Instead, the Universe is so hot and dense that what we have is known as a quark-gluon plasma. The reason for this is counterintuitive, especially if the only forces you’re familiar with are gravity (obeying Einstein’s general relativity) and electromagnetism (obeying Maxwell’s equations classically and the science of quantum electrodynamics in the quantum world). Under those more familiar circumstances, the forces get stronger in magnitude the closer you bring two particles. Halve the distance between two electric charges and the force quadruples between them; halve the distance between two masses and the force might even more-than-quadruple, as general relativity dictates.
But this is absolutely not how the strong nuclear force works at all. If you were to take two quarks, antiquarks, or a quark-antiquark combination, for example, and halve the distance between them, you would find that the strength of the strong nuclear force that binds them together does something very different.
It doesn’t quadruple.
It doesn’t even double.
Instead, the force between them drops, and it drops to smaller and smaller values the closer together you bring your quarks. (Or, for that matter, any other particle with a color-charge that exists.)

This seems weird, doesn’t it? Nevertheless, this is how the insides of all atomic nuclei, and the strong nuclear force in general, actually works. There’s a simple analogy that we’re more familiar with that might make more sense: a spring. When you have two masses attached to either end of an unstretched spring, the spring exerts no force on them; the lack of stretching means there’s no force pulling them back together. On the other hand, the greater the amount you stretch the spring by, the greater the force exerted on each of the masses at the ends, pulling them back together. This is extremely similar to how the strong nuclear force behaves.
Below a certain distance, the force between any two particles with a color-charge (quarks and gluons) actually drops to zero, only increasing as they get farther apart. At the high temperatures and densities present at these very early times, the nuclear force is too weak to bind anything together: acting like a series of unstretched springs. As a result, particles simply zip around, colliding with each other, creating new ones and annihilating away. But as the Universe expands, it both cools and gets less dense. And as time goes on, it becomes harder to make the more massive particles, and the typical distance between them increases.

In addition, with the exception of the lightest quarks (up and down, plus anti-up and anti-down) and the lightest charged lepton (the electron, plus the positron), all the other charged particles of the Standard Model are unstable against radioactive (weak) decays. As the clock after the start of the hot Big Bang continues to tick away, picoseconds turn into nanoseconds, and the nanoseconds pile up into microseconds. As this process continues, the heavier particles stop being created and slowly disappear from our Universe.
Top quarks were gone as soon as the electroweak symmetry broke, decaying away in mere yoctoseconds. Bottom/anti-bottom quarks disappear next, followed by the tau and antitau leptons. After that, the charm/anticharm quarks go, followed by the strange/antistrange quarks. After perhaps 100 nanoseconds have gone by, only the up-and-down quarks (and antiquarks), electrons and positrons, muons and antimuons, and the neutrinos and antineutrinos remain among the massive particles. And yes, photons, gluons, and gravitational waves still persist during these times as well.

As the Universe cools, it becomes less diverse in terms of the number of species of particles that can exist within it. As we lose more and more particle/antiparticle combinations, they create greater numbers of the lighter particle/antiparticle pairs that can still exist, including greater numbers of photons. And these photons, because they’re perfectly stable, never go away, but simply persist for all time.
Each and every time we produce two photons from particle/antiparticle annihilation, it slows down the cooling of the Universe a little bit. The Universe is getting cooler and sparser, but it’s also changing in terms of what exists within it. In the early stages, only a small-but-substantial percentage of the particles around are photons, neutrinos, and antineutrinos. But as these heavier charged particles start to disappear, like quarks-and-antiquarks and leptons-and-antileptons, the fraction of energy in photons, neutrinos, and antineutrinos continues to rise higher and higher.
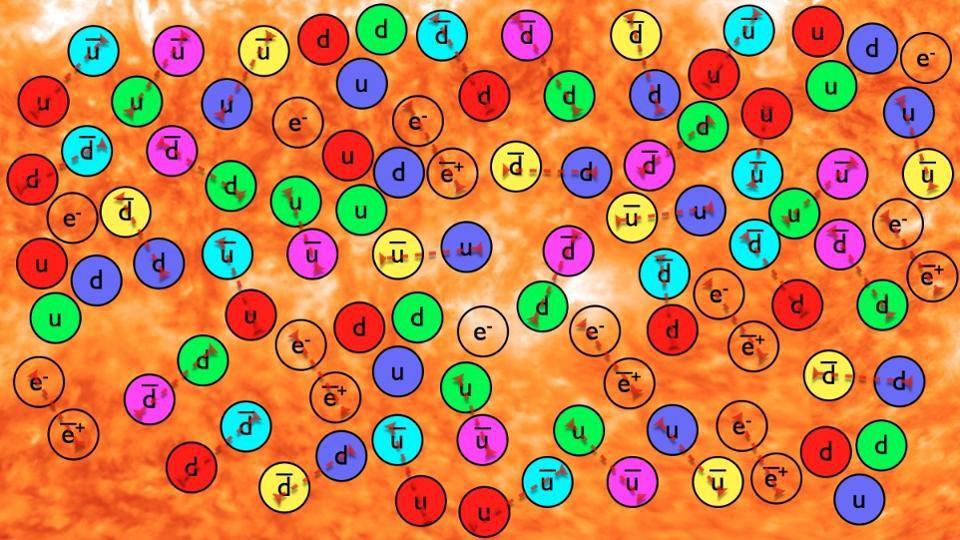
This process occurs rapidly, and time waits for no particle or antiparticle. As time continues to march on, and hundreds-of-nanoseconds add up to become microseconds, the Universe cools down even more severely. After a few microseconds go by, the muons and antimuons start to decay away, and at the same time, the average distance between particles (remember, the Universe is still expanding!) continues to increase.
Right about this time, the up-and-down quarks (plus the anti-up and anti-down quarks) start to separate away to substantial (femtometer: 10-15 m) distance scales. This number is important, because femtometer scales are about the scale at which the spring-like strong force begins to get substantial. About 10-to-20 microseconds after the Big Bang, we hit a critical temperature/density combination. We’ve now cooled down to a temperature of around 2 trillion K (2 × 1012 K), and at this point, the quarks and antiquarks are far enough apart that the strong force starts to become a very big deal indeed.
Just like an unstretched spring doesn’t exert a force but a stretched spring does, the quarks don’t feel a confining force until they reach a certain distance. But once they do, they become bound.
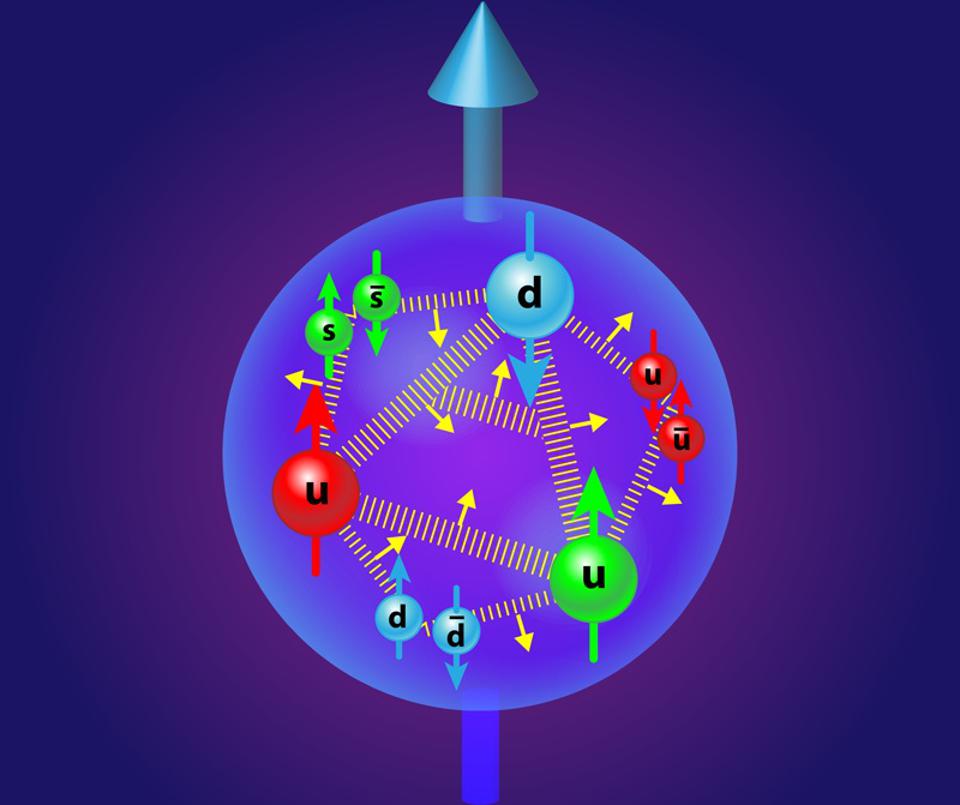
Gradually, the Universe undergoes a critical transition: from free up, down, anti-up, and anti-down quarks to bound protons, neutrons, antiprotons, and antineutrons. What was once a quark-gluon plasma has now passed through what’s known as the era of confinement, as temperatures and densities become low enough that free, unbound quark states can no longer stably persist. The Universe is still hot enough to make new particle-antiparticle combinations, and created plenty of up/anti-up and down/anti-down quark combinations when things were dense enough: producing a brief “rain” of mesons (such as pions) in the early, microsecond-aged Universe.
But as the Universe continues to expand and cool, both temperatures and densities drop. Once those temperatures and densities drop to low enough values, we’ll have stable protons and neutrons (and antiprotons and antineutrons) instead. At this epoch, just tens of microseconds after the hot Big Bang, the Universe isn’t hot enough to spontaneously create new proton/antiproton or neutron/antineutron pairs. What this means is that when protons and antiprotons (or neutrons and antineutrons) find each other, they annihilate away, and no additional new ones are spontaneously created.

What happens, then, as the Universe cools through this critical stage where a quark-gluon plasma transitions into forming bound states (hadrons, in particle physics terminology) is as follows.
- Any remaining free quarks begin to experience confinement, becoming protons, neutrons, antiprotons, antineutrons, and pions (the lightest example of the unstable particles known as mesons).
- The mesons rapidly decay away, while the antiprotons and antineutrons annihilate with the protons and neutrons, with other collisions unable to create new ones.
- Which then leaves us with protons and neutrons alone, only because at some earlier stage, the Universe created more matter than antimatter.
The number of excess protons and neutrons is small, as the level of the matter-antimatter asymmetry is just below 1-part-in-1,000,000,000 (one in a billion). Nevertheless, this asymmetry is tremendously important, as it means that as we transition from a quark-gluon plasma Universe to one with confined hadrons, we aren’t just left with “nothing” (or nearly nothing) after they all annihilate away. That asymmetry, created a few steps earlier in the story of the hot Big Bang, now persists, and will eventually become incredibly important down the road.

At last, the Universe starts to resemble something we’d recognize today. Sure, it’s hot and dense. Sure, there are no atoms or even any atomic nuclei. Sure, it’s still filled with a bunch of positrons (the antimatter counterpart of electrons) and electrons, and is still creating-and-annihilating them spontaneously. But most of what exists at this early moment, perhaps 25 microseconds after the start of the hot Big Bang, still exists in some form today, 13.8 billion years down the line.
The protons and neutrons will become the building blocks of atoms; the neutrinos and antineutrinos and photons will become part of the cosmic background; the leftover electrons that will exist when the electron/positron pairs annihilate away will combine with the atomic nuclei to make atoms, molecules, and complex biochemical reactions possible.
But at this stage, the biggest occurrence is that particles are no longer individual-and-free on all scales. Instead, for the first time, the Universe has created a stable, bound state of multiple particles. A proton is two up and one down quark, bound together by gluons, while a neutron is one up and two down quarks, bound together by gluons.
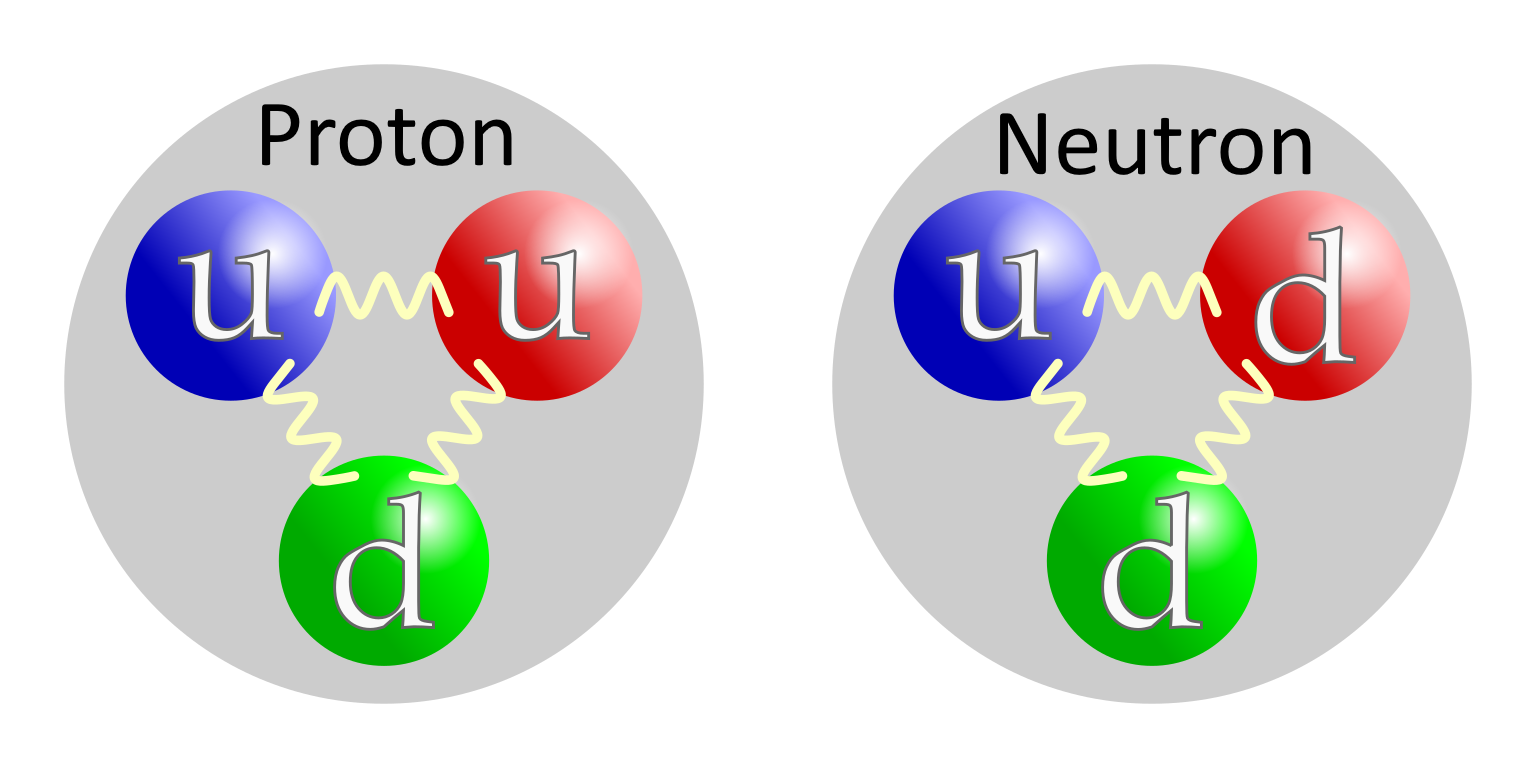
It’s only because we created more matter than antimatter that we wind up with a Universe that has protons and neutrons left over. And that, itself, is only possible because the Higgs gave rest mass to the fundamental particles (i.e., quarks) that make them up. If everything were massless, or if everything were matter-antimatter symmetric, there would be no bound, stable atomic nuclei that could persist.
Owing to the nature of the strong force, and the tremendous binding energy that occurs in these stretched-spring-like interactions between the quarks, the masses of the proton and neutron are some 100 times heavier than the quarks that make them up. The Higgs gave mass to the Universe, but confinement is what gives us 99% of our mass. Without protons and neutrons, the Universe we inhabit today wouldn’t be possible, and complex entities that require the existence of atoms, like us, could never have existed at all.