Pulsars, not dark matter, explain the Milky Way’s antimatter
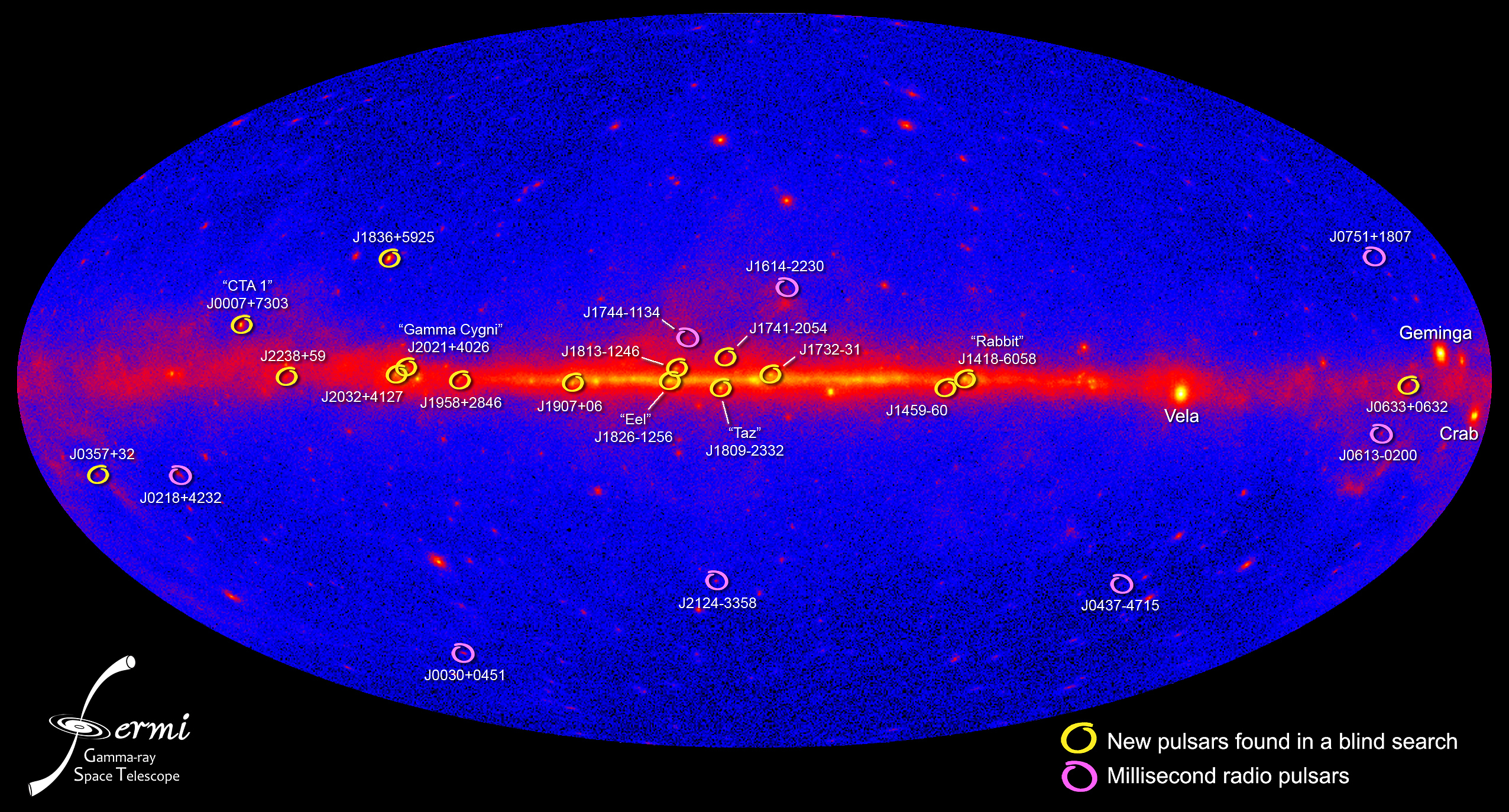
- For years, astronomers have been puzzled by an excess of antimatter particles emanating from the Milky Way’s galactic center.
- Many astronomers and physicists hoped that this phenomenon would’ve been caused by dark matter particles, either annihilating or decaying, and then creating positrons.
- However, recent results suggest a far more mundane explanation: rotating neutron stars, whose relativistic jets and fast-moving material create matter-antimatter pairs. No dark matter required.
When you look out at the Universe, what you see is only a tiny portion of what’s actually out there. If you were to examine the Universe solely with what’s perceptible to your eyes, you’d miss out on a whole slew of information that exists in wavelengths of light that are invisible to us. From the highest-energy gamma rays to the lowest-energy radio waves, the electromagnetic spectrum is enormous, with visible light representing just a tiny sliver of what’s out there. At shorter wavelengths and higher energies, gamma rays, X-rays, and ultraviolet light are all present, while at longer wavelengths and lower energies, infrared, microwave, and radio light encodes a wide variety of information about what various astrophysical sources are doing.
However, there’s an entirely different method to measure the Universe: to collect actual particles and antiparticles, a science known as cosmic ray astronomy. For more than a decade, astronomers have seen a signal of cosmic ray positrons — the antimatter counterpart of the electron — that they’ve struggled to explain. Could it be humanity’s best clue toward solving the dark matter mystery? While many hoped that the answer would be “yes,” a recent study definitively says no, it’s probably just pulsars. Here’s why.

There are a great many things in the Universe that are known to create positrons: the antimatter counterpart of electrons. Whenever you have a high-enough energy collision between two particles, there’s a certain amount of energy that will be available with the potential to create new particle-antiparticle pairs. If that available energy is greater than the equivalent mass of the new particle(s) you want to create, the available energy as defined by Einstein’s famous equation, E = mc², there will be a finite probability of generating those new particles with every such collision that occurs.
There are all sorts of high-energy processes that can lead to this type of energy becoming available, including:
- particles accelerated by black holes,
- high-energy protons colliding with the galactic disk,
- or particles accelerated in the vicinity of neutron stars.
However, it’s also possible that they could arise from an exotic source, such as dark matter or a different sort of new particle from beyond the Standard Model. Based on the known physics and astrophysics of the Universe, however, we know that a certain amount of positrons must be generated irrespective of any new physics. “New physics” should only be considered if the amount of positrons generated by the more mundane physical mechanisms is shown to be insufficient.

However, we also expect that there actually must be some new physics out there, because of the overwhelming astrophysical evidence for dark matter. While the true nature of dark matter will remain a mystery until the particle (or at least one of the particles) responsible is detected directly, many dark matter scenarios exist where not only is dark matter its own antiparticle, but that dark matter annihilations will also produce electron-positron pairs. As long as your dark matter particle is massive enough so that electron-positron pairs can be generated from the energy released when dark matter particles annihilate, this type of antimatter ought to be generated under some conditions.
So how do we decide: dark matter or “mundane” physics? Which one is responsible for blowing these “Fermi bubbles” in our galaxy?
Whenever you have multiple possible physical explanations for what could cause an observable phenomenon, the key to telling which one matches reality is to tease out differences between the explanations. In particular, positrons due to dark matter should experience a cutoff at specific energies (corresponding to the mass of the dark matter particles), while positrons generated by conventional astrophysics should fall off more gradually. In other words, looking at the energy spectrum of these positrons, directly, should tell us what the answer is.
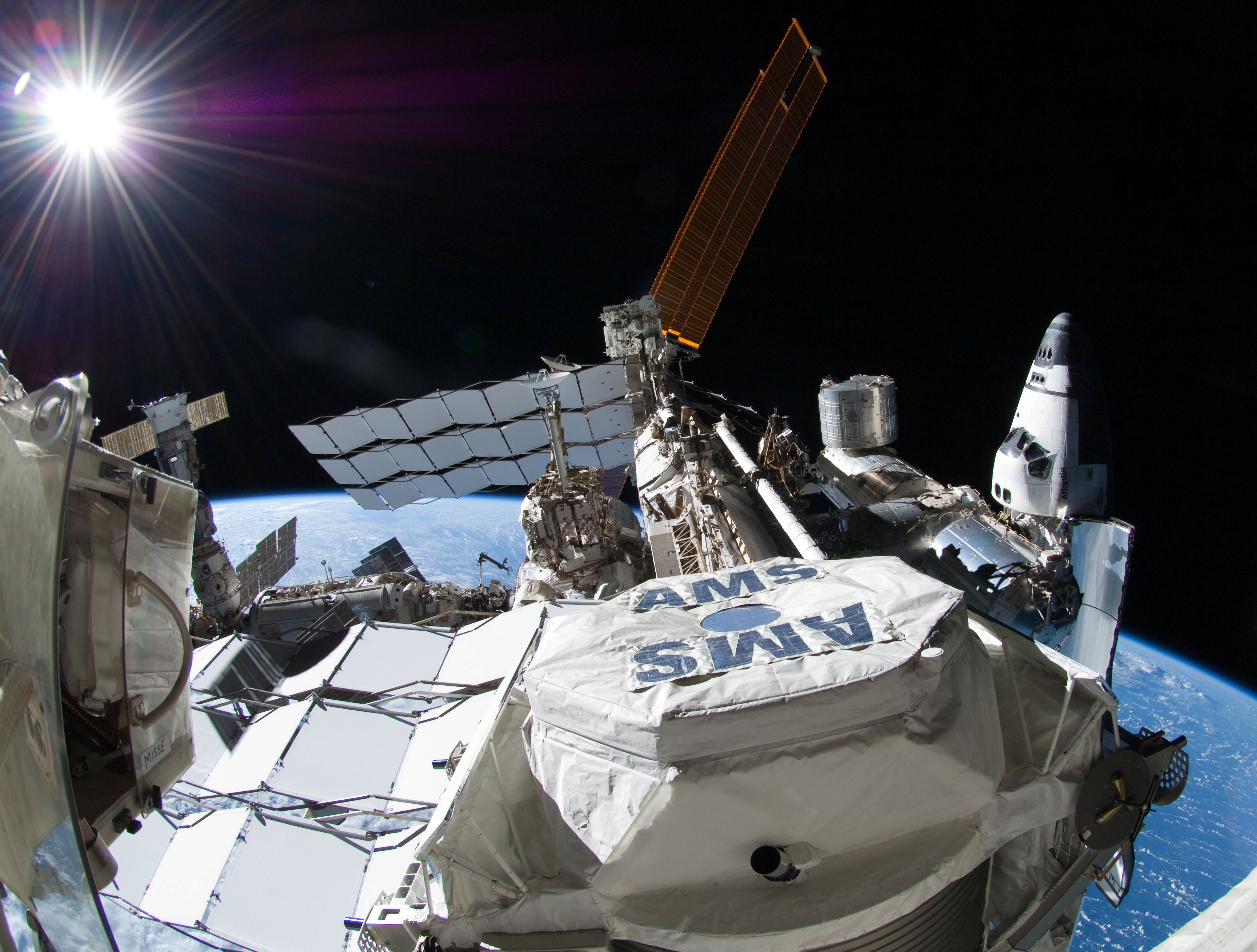
In 2011, the Alpha Magnetic Spectrometer experiment (AMS-02) was launched, with the goal of further investigating this mystery. After arriving at the International Space Station aboard the final mission of the Space Shuttle Endeavor, it was quickly set up and began sending data back to Earth within 3 days. During its operational phase, it collected and measured more than ten billion cosmic ray particles per year. Although only a tiny fraction of them were positrons, that’s okay; because of the different energy, charge, and charge-to-mass ratios of the different particles that impacted it, different species of particle could be separated out.
What’s remarkable about AMS-02 is the “magnetic” part of the experiment: magnetic fields bend charge particles, and while the magnetic force on a moving charged particle depends only on its electric charge and velocity, the amount that a particle “bends by” as it passes through the detector is also dependent on the incoming particle’s mass and momentum. This makes AMS-02 able to sort these cosmic rays both by type and by energy, providing us with an unprecedented set of data to evaluate whether the positrons appeared to be due to dark matter or not. At low energies, the data matched the predictions of cosmic rays colliding with the interstellar medium, but at higher energies, something else was clearly at play.
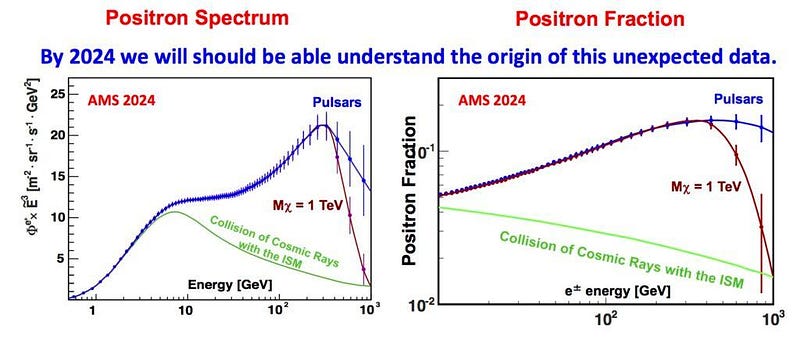
However, just because you’re seeing more positrons above and beyond what we expect would be caused by collisions of cosmic rays with matter in the interstellar medium doesn’t necessarily mean it’s a slam dunk for dark matter. That’s not true by any means. At higher energies, it’s also possible that pulsars, which accelerate matter particles to incredible energies through a combination of their gravitational and electromagnetic forces, could produce a peaked excess of positrons at high energies.
The only way to truly tell those scenarios apart is to look at the highest energies, and see whether the energy-spectrum fall-off is sharp (for dark matter) or gradual (for pulsars): something right at the limit of AMS-02’s capabilities. Although AMS-02 sees evidence (at 4-sigma, or 99.99% confidence) that there’s a peak and then a falloff in the observed energies of positrons, its sensitivity and event rate peters out at exactly the types of energies that would enable us to differentiate between a positron signal arising from pulsars versus one arising from annihilating dark matter.
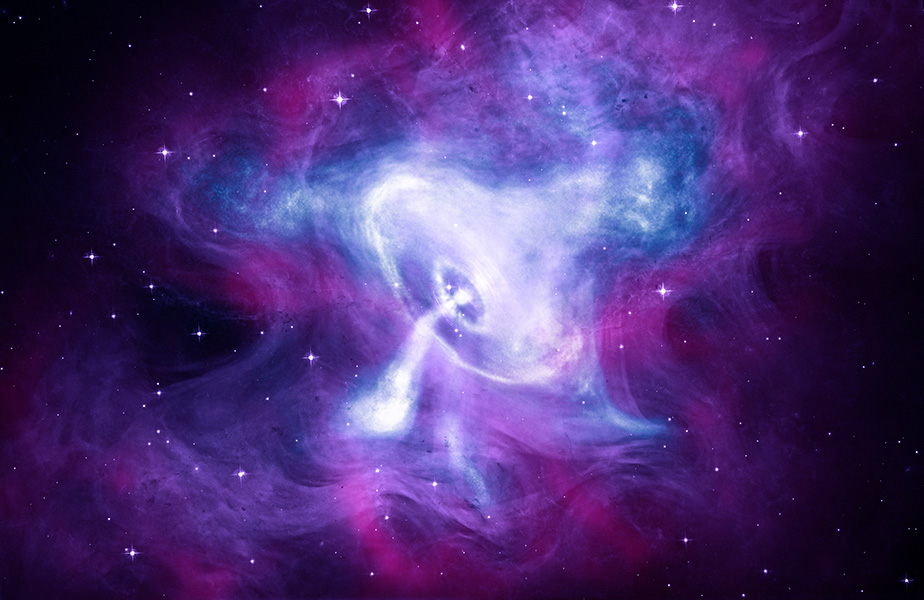
However, there’s more than one way to tell these two scenarios apart, as positrons produced by pulsars should also generate an additional signal that falls well outside the measurements that AMS-02 or any cosmic ray experiment could detect: gamma rays. In other words, there’s not only a direct “antimatter” signature that we should be attempting to observe, but to search for a high-energy electromagnetic signature — and remember, gamma-rays are the highest energy electromagnetic waves of all — right alongside it. There’s a physical reason behind this explanation.
If it’s pulsars that are truly generating the positrons that could be responsible for the signal that cosmic ray experiments are seeing, then a significant fraction of those positrons will have the misfortune of colliding with electrons in the interstellar medium long before they arrive at our cosmic ray detectors. When positrons collide with electrons, they annihilate, with each reaction producing two gamma rays with a very specific energy signature: 511 keV of energy, the rest-energy equivalent of an electron’s (or positron’s) mass, also obtained from Einstein’s E = mc².

However, pulsars should theoretically be able to accelerate these electrons and positrons up to extraordinarily high energies: energies that even the world’s most powerful terrestrial particle accelerator, the Large Hadron Collider, is wholly incapable of reaching. When photons — even those containing only the normal-energies that starlight produces — interact with these ultra-relativistic (near light-speed) particles, they can get boosted to extraordinary energies through a process known as inverse Compton scattering.
Based on physical parameters like:
- the properties of the pulsar,
- the matter in the pulsar’s vicinity,
- the electrons and positrons generated,
- and the amount of starlight present nearby,
a specific energy spectrum will be created for the photons generated from this process. If you combine the energy spectra, all superimposed together, from all of the nearby, relevant pulsars, you’ll predict a specific gamma ray signature that you expect to observe. That signature is what could indicate whether it’s pulsars, or whether it’s something that’s not pulsars (like dark matter), that are causing the observed positron excess.
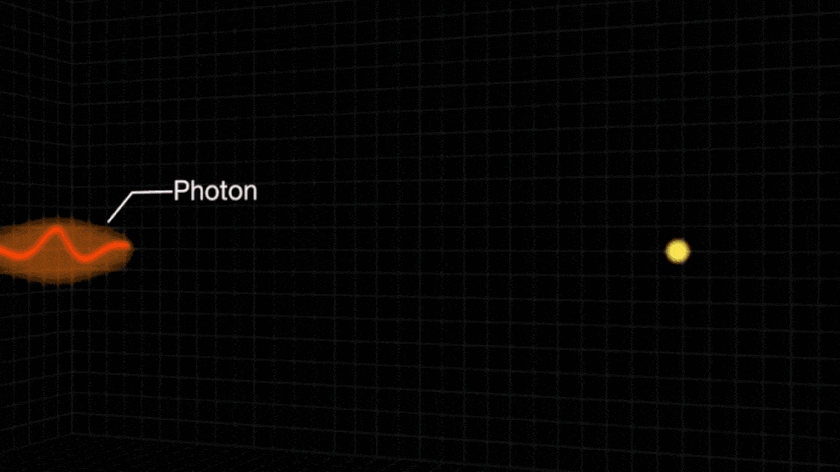
About 800 light-years away, incredibly close by astronomical standards, one of the brightest gamma-ray pulsars in the entire sky can be found: Geminga. This pulsar was only discovered in 1972, and had its nature revealed in 1991, when the ROSAT mission measured evidence for a neutron star spinning at a rate of 4.2 revolutions-per-second.
Fast-forward to the present day, more than 30 years later, when we now have NASA’s Fermi Large Area Telescope: with enormously improved spatial and energy resolution over any gamma-ray observatory that preceded it. Fermi-LAT is now the world’s most sophisticated gamma ray observatory by many metrics, and it’s capable of observing details about individual sources, such as pulsars, that couldn’t be seen before.. By subtracting out the gamma ray signal arising from cosmic rays colliding with interstellar gas clouds, the remnant signal from starlight interacting with accelerated electrons and positrons could be revealed.
When a team of researchers led by Mattia di Mauro analyzed the relevant Fermi data in late 2019, what they saw was spectacular: an energy-dependent signal that, at its largest, spanned some 20 degrees in the sky at the exact energies that AMS-02 was most sensitive to.
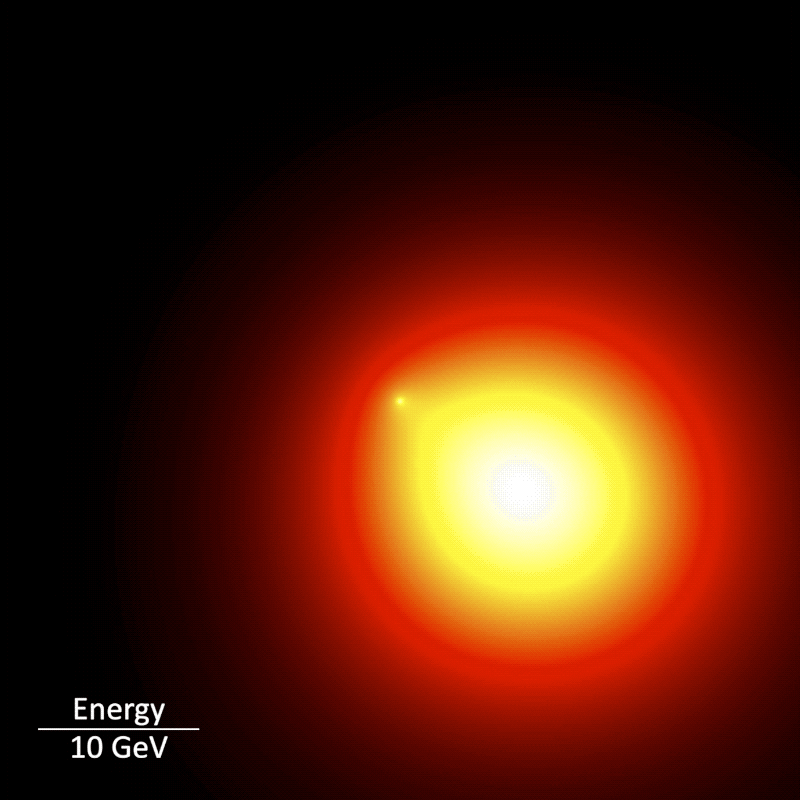
Explaining this glow, which decreases in size as Fermi looks at progressively higher energies, fit the models perfectly by leveraging a combination of inverse Compton scattering with the pulsar’s motion through interstellar space. According to Fiorenza Donato, coauthor on the recent Fermi study that measured gamma rays from Geminga,
“Lower-energy particles travel much farther from the pulsar before they run into starlight, transfer part of their energy to it, and boost the light to gamma rays. This is why the gamma-ray emission covers a larger area at lower energies. Also, Geminga’s halo is elongated partly because of the pulsar’s motion through space.”
This makes sense if you remember an important aspect of photon-electron scattering, the process that powers the “boost” to gamma-ray energies: higher energy particles have a greater cross-section with photons, and so are more likely to interact within a shorter distance.
The measurement of the gamma rays arising from Geminga, alone, suggests that this one pulsar could be responsible for as much as 20% of the high-energy positrons that were seen by the AMS-02 experiment.
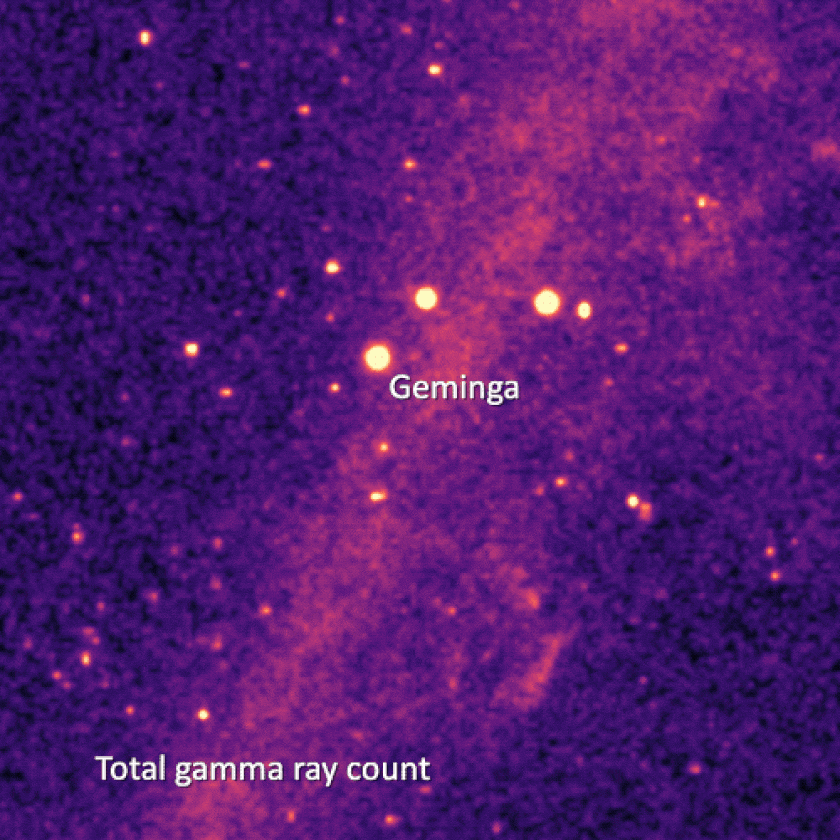
Whenever there’s an unexplained phenomenon that we’ve measured or observed, it presents a tantalizing possibility to scientists: that perhaps there’s something new at play beyond what’s presently known. We know there are mysteries about our Universe that require new physics at some level — mysteries like dark matter, dark energy, or the cosmic matter-antimatter asymmetry — whose ultimate solution has yet to be discovered. It’s an important reminder that all of the known physics at play in the Universe cannot represent all of what’s truly out there; there still remain deep, fundamental mysteries whose solutions are not presently known.
However, we cannot claim evidence for a new discovery until we first scrupulously and quantitatively account for everything that represents the physics and astrophysics of what’s already known. By factoring in the effect of pulsars, the positron excess observed by the Alpha Magnetic Spectrometer collaboration appears to be explicable entirely by conventional high-energy astrophysics, with no need for dark matter. Right now, our observations are consistent with the notion that pulsars are responsible for 100% of the observed excess, requiring scientists to go back to the drawing board for a direct signal that reveals our Universe’s elusive dark matter. The Milky Way’s antimatter, despite what many had hoped for, appears to be entirely due to pulsars.