The Universe might never run out of hydrogen
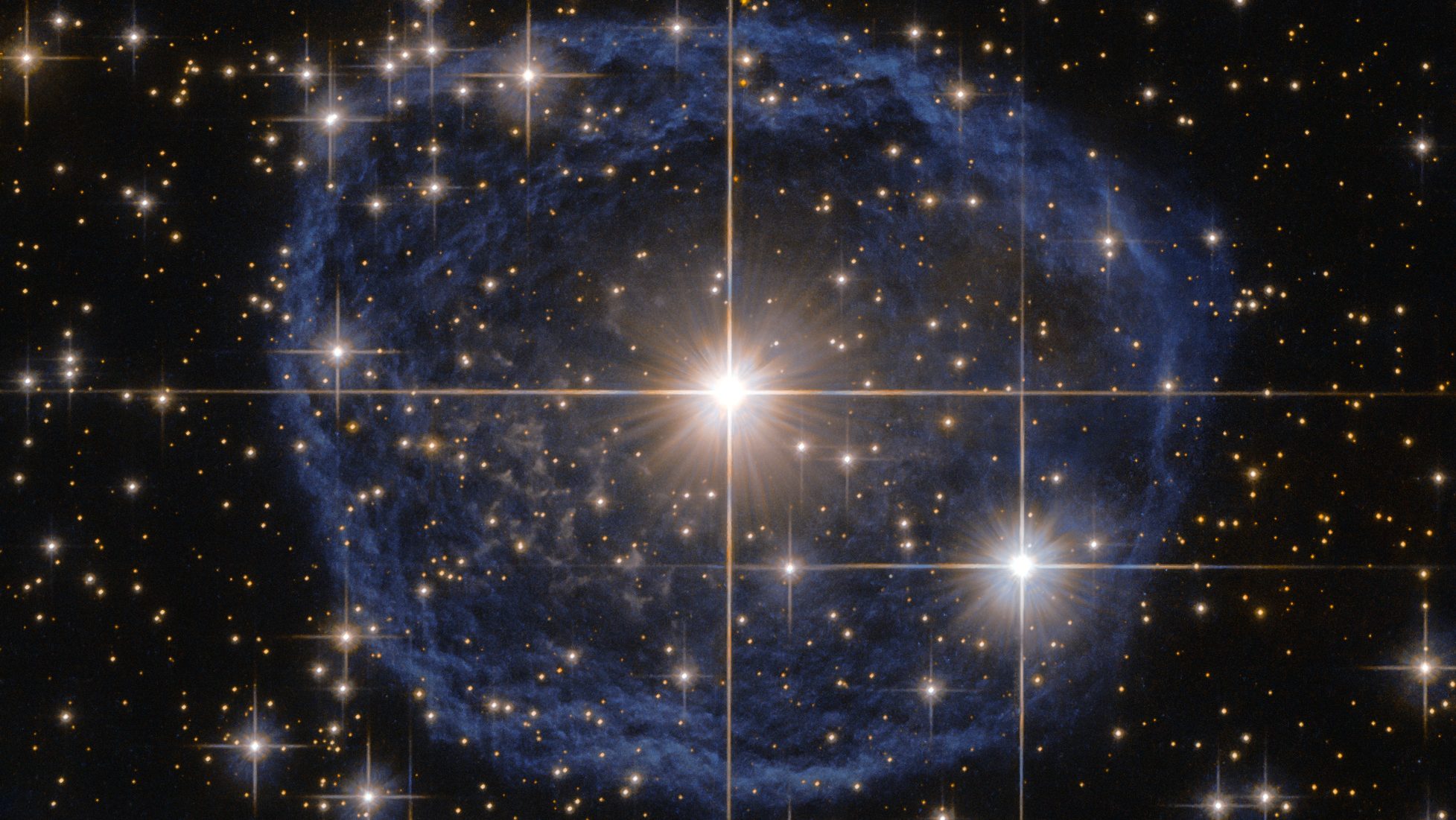
- The most common element in the Universe, by both number and mass, is hydrogen: a fact that was true immediately after the Big Bang and that remains true even today.
- But the main nuclear fusion process that powers the stars is the fusion of hydrogen into helium, increasing the abundance of heavier elements at the expense of hydrogen.
- After enough time goes by and enough stars have formed, does that mean we’ll run out of hydrogen, and no further star-formation will be possible? Let’s find out.
Nothing in this Universe lasts forever, no matter how large, massive, or enduring it appears to be. Every star that’s ever born will someday run out of fuel in its core and die. Every galaxy that’s actively forming stars will someday run out of star-forming material and cease doing so. And every light that shines will someday cool off and go dark. If we wait long enough, there will be nothing to see, observe, or even extract energy from; when it reaches a state of maximal entropy, the cosmos will achieve a “heat death,” the inevitable final-stage in our cosmic evolution.
But what, exactly, does that mean for the simplest atom of all: hydrogen, the most common element in the Universe since the start of the Big Bang? That’s what Bill Thomson wants to know, writing in to ask:
“I read somewhere that eventually all the hydrogen in the universe will be consumed and will no longer be available to fuel the stars. Presumably all the hydrogen will be used up in the furnaces of the multi-trillions of stars. Do you think this is possible?”
It’s possible, but whether or not that will ever happen is open to both debate and interpretation. Here’s the story — past, present, and future — of the simplest, most common elements of all.
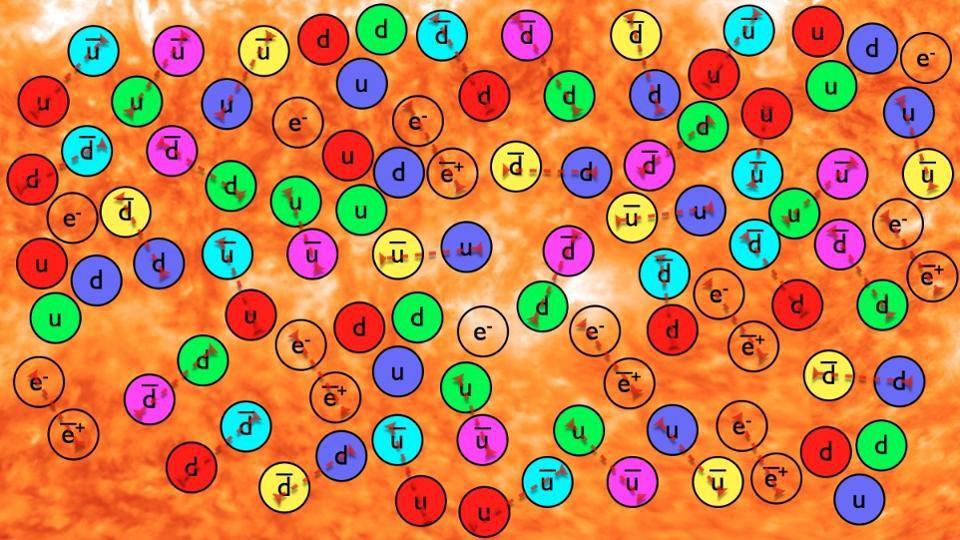
The past
The most common element in our Universe today is hydrogen, just as it was in the immediate aftermath of the hot Big Bang. What’s remarkable is that it didn’t have to turn out this way; if things had been only somewhat different, we would’ve began with a Universe that had practically no hydrogen at all, and where helium was the lightest element available.
The reason things turned out the way they did — where 92% of the atoms (by number) and 75% of the elemental composition (by mass) of the Universe was hydrogen, even before the formation of any stars — was due to the radiation content of the Universe in the immediate aftermath of the Big Bang.
The reason isn’t intuitive, but it is at least straightforward. In the very early Universe, shortly after the hot Big Bang, the Universe consisted of all the particles and antiparticles that it’s possible to create, as there was enough energy available in every collision of two quanta to spontaneously bring particle-antiparticle pairs into existence of all types via Einstein’s E = mc². It was only as the Universe expanded and cooled, and the corresponding energy-per-quantum dropped, that the heavier, unstable particles (and antiparticles) annihilated and/or decayed away.
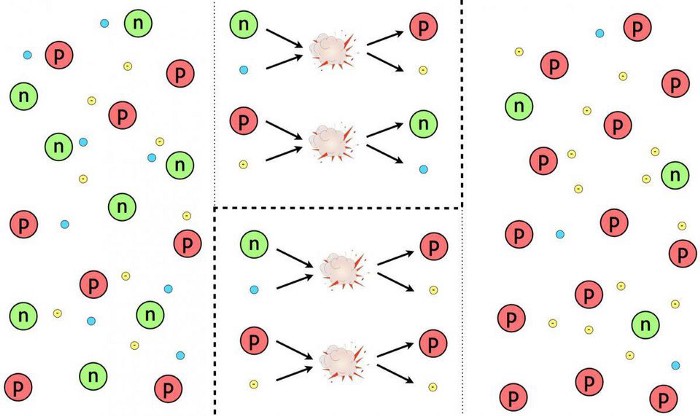
Eventually, a few microseconds after the start of the hot Big Bang, quarks and gluons transitioned from a plasma into bound states: primarily protons and neutrons, coexisting in a roughly 50/50 split. The proton/neutron ratio remains at about a 50/50 split for about a few tenths of a second in our Universe, as protons and neutrons interconvert at equal rates, with protons and electrons fusing to become neutrons and neutrinos (and vice versa), and protons and antineutrinos fusing to become neutrons and positrons (and vice versa).
But then, three processes compete, vying for dominance, with the winner dependent on the conditions within our Universe.
- The energy-per-particle drops low enough, as the Universe expands, so that neutrons interacting with either positrons or neutrinos have enough energy to convert to protons, but only a fraction of protons interacting with either electrons or antineutrinos have enough energy to convert into neutrons.
- Free neutrons, which are unstable with a half-life of around 10 minutes, radioactively decay into protons (plus an electron and an antineutrino).
- Nuclear fusion occurs between protons and neutrons, building up a chain that rapidly leads to the formation of helium-4: with two protons and two neutrons in its nucleus.

Perhaps surprisingly, there’s only one major factor that determines what the elemental abundance is going to wind up being just prior to the formation of any stars: the ratio of photons to baryons (i.e., protons and neutrons combined) at this stage. If there are only a few photons for each baryon, then that third factor — nuclear fusion between protons and neutrons — will proceed very early and very rapidly, giving you a Universe whose atoms are made of ~100% helium (or heavier) and ~0% hydrogen. Similarly, if there are too many photons per baryon (like 1020 or more), then the second factor of neutron decay dominates, and the Universe will be almost exclusively hydrogen before nuclear fusion can stably occur; too many photons will blast the first fragile step in nuclear fusion (deuterium) apart.
But in our Universe, where we have just over one billion (109) photons per baryon, all three processes matter. The neutron-proton interconversion scales tip as the Universe cools, leading to protons outnumbering neutrons by about 5:1 after a few seconds. Then, that process becomes inefficient, and neutrons decay over the next 3.5 minutes or so, resulting in a proton-to-neutron ratio of about 7:1. Finally, nuclear fusion occurs, and that gives us a Universe that’s about 75% hydrogen and 25% helium-4 by mass, or 92% hydrogen and 8% helium by number-of-atoms. That fraction persists for millions of years, until the first stars begin to form.

The present
It’s now been 13.8 billion years since the Big Bang, and our observable Universe has expanded and cooled the entire time. It’s also gravitated, and the densest gravitational clumps have grown into massive structures, rich in stars and galaxies. All told, if we sum up the number of stars formed within our observable Universe over that time, it comes out to a couple of sextillion, and all the nuclear fusion that’s occurred has shifted the atomic balance in our Universe by quite a bit. By mass, today, our Universe is now approximately:
- 70% hydrogen,
- 28% helium,
- 1% oxygen,
- 0.4% carbon,
- and about 0.6% everything else combined, led by neon, then iron, nitrogen, silicon, magnesium, and sulfur.
By number, however, hydrogen still dominates, still making up approximately 90% of all atoms in the Universe. Despite all the star-formation that’s occurred — and there’s been an enormous amount of it — almost all of the atoms in the Universe are still plain old hydrogen, with just one proton for its nucleus.
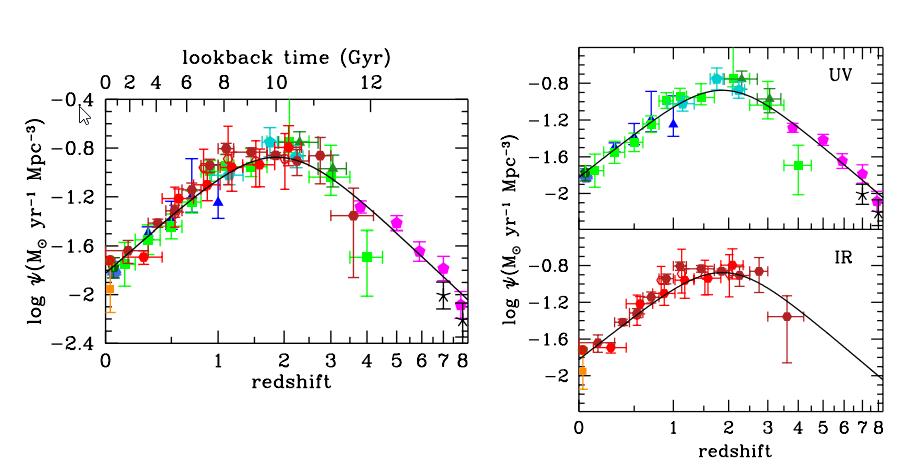
You might think, therefore, that we have a long, long way to go before the Universe runs out of hydrogen. But there’s another piece to the puzzle that suggests maybe “running out of hydrogen atoms” isn’t the problem we might intuit: the star-formation history of the Universe. Here in the Milky Way, one of trillions of galaxies within the observable Universe, we’re forming about 0.7 solar masses worth of new stars each year: a paltry amount. It’s not particularly paltry compared to a typical galaxy; based on the Milky Way’s mass, gas content, and the proximity of nearby galaxies, its star-formation rate is right in line with what typical galaxies within our Universe are doing at this moment in time: 13.8 billion years after the Big Bang.
But this is a paltry amount of star-formation compared to what the Universe was doing billions of years ago. In fact, the current star-formation rate, overall, is just 3-5% of what it was at its peak some ~11 billion years ago. Star-formation reached its maximum rate way back then, and has been steadily decreasing ever since. There’s no indication that this decrease is going to stop anytime soon, either; as far as we can tell — although there will be localized bursts of new star formation, including right here, when the Milky Way and Andromeda merge some 4 billion years in the future — the star-formation rate should continue to drop further and further as time goes on.
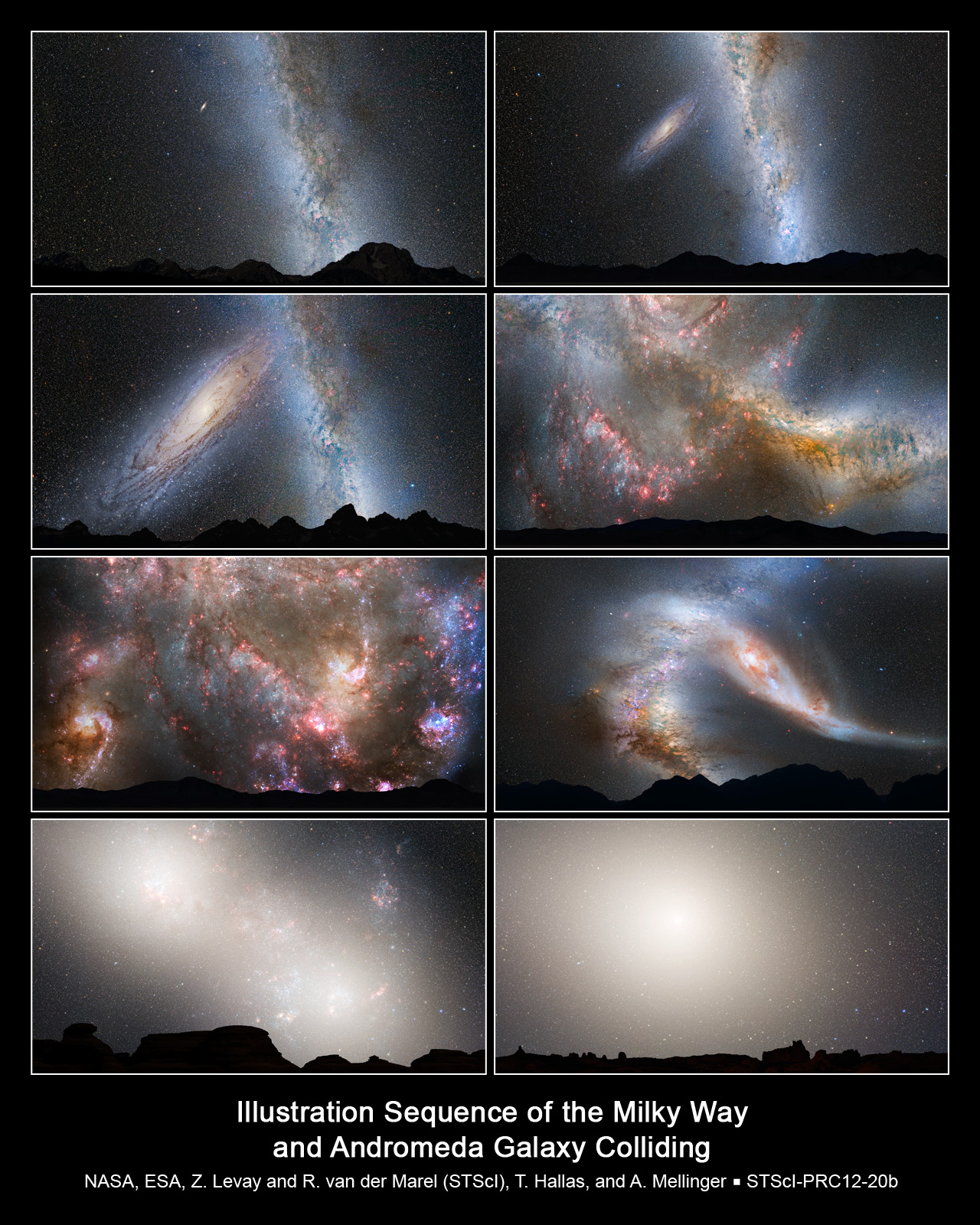
Part of the reason for this decrease is that as galaxies evolve, they do things like:
- undergo bursts of star-formation,
- speed through the intra-group and intra-cluster medium,
- and experience tidal interactions from galactic neighbors,
which are all examples of events that cause gas to get stripped out of or ejected from the host galaxy. Many of the galaxies that exist at the centers of rich galaxy clusters are already what we call “red and dead,” not because of some astronomical penchant for anti-communist propaganda but because, without enough gas to form new generations of stars, the high-mass, short-lived blue stars die out, leaving only the lower-mass, longer-lived, lower-brightness, redder-in-color stars behind.
In a galaxy like our own, where we’ve been lucky enough to live in relative isolation and are still gas-rich, future mergers will lead to major new episodes of star-formation, which in turn will eject a substantial portion of our galaxy’s gas into intergalactic space: beyond the gravitational pull of our Local Group. We’ll end up in a gas-depleted state, but even though the rate of star-formation will drop, it shouldn’t cease entirely. We should expect to see new, ongoing star-formation not only for billions of years to come but many trillions of years. The big open question, however, is how much star-formation, overall, remains.
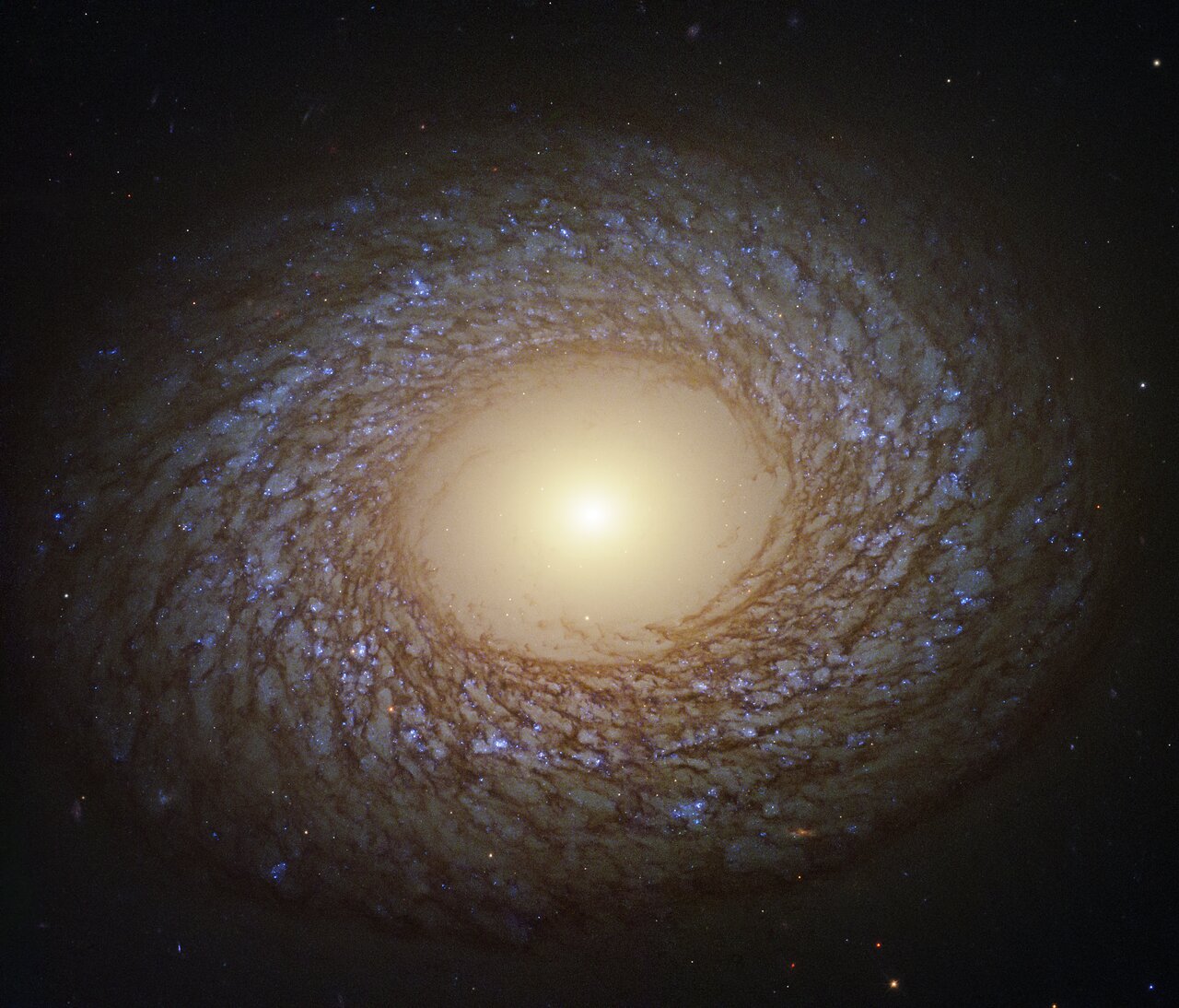
The future
One of the key realizations of astronomy in recent decades is how surprisingly inefficient star-formation actually is at consuming and using up hydrogen gas. If you begin with a massive molecular cloud of gas and it contracts down to form a large number of new stars — say, hundreds, thousands, or even greater numbers of stars — it turns out that only about 5-10% of the gas goes into the newborn stars. The remaining 90-95% gets gently blown back off into the interstellar medium by a combination of radiation and stellar winds, where it can eventually participate in future generations of star-formation.
Additionally, while most of the stars that form, by number, will be low-mass, long-lived red dwarf stars that will fully convect and end up fusing all of their hydrogen into helium, most of the stars that form by mass won’t do so; they’ll only fuse the hydrogen in their cores into helium or heavier elements. The outer layers, whether the star dies violently in a supernova or peacefully in a planetary nebula, will get ejected and, once again, returned to the interstellar medium. When it comes to the deaths of Sun-like stars, most of the hydrogen that composed their outer layers go right back into space, where they’ll have star-forming potential once again.

In other words, it likely won’t be the consumption of hydrogen by nuclear fusion processes that brings an end to star-formation; according to most of the simulations and calculations we can perform, the majority of atoms in the Universe always has been and always will be simple hydrogen atoms. The star-formation rate will drop, but as long as galaxies maintain a sufficient reservoir of hydrogen gas, then when gravitational contraction occurs in massive enough clumps, new stars can still form. This might not lead to a very large number of new stars compared to what’s already been formed, but star-formation should persist for at least 100 trillion years into the future.
But what will happen, especially once enough time has passed, is that gravitational interactions will eject matter of all types — stars, planets, and even individual atoms and particles — from their host galaxies. Whenever you have gravitational interactions between many objects of many different masses in dense environments, the more massive, denser objects tend to sink to the center, while the less massive, lower density objects tend to get ejected. On timescales of quadrillions of years and up, this process will dominate, ejecting any remnant quantities of gas from galaxies that might remain.

A long time into the future, there won’t be new episodes of star-formation to bring new sources of light into existence any longer. All we’ll have to rely on are the occasional, random mergers of brown dwarfs — failed stars of less than 0.075 solar masses — crossing that critical mass threshold to initiate nuclear fusion and bring new stars to life. These events will be rare, but should enable a trickle of new stars, where hydrogen gets converted to helium in their cores, to form until the Universe is around 1021 years old or so. Beyond that point, gravitational ejection should become efficient enough so that only stellar corpses remain within any remnant galaxies, including our own.
But even at the very end of all of this, countless years into the future, we should still be able to draw an imaginary sphere around what comprises our visible Universe today and count the atoms inside. If we did, we’d find that somewhere around 85-88% of those atoms were still hydrogen atoms by number, it’s just that most of them would be found roaming the depths of empty, intergalactic space, too sparse and too isolated to ever form stars again. The Universe may someday become cold, empty, dark, and starless, but it won’t be for a lack of hydrogen!
Ethan is on medical leave until May 6th. Please enjoy a republication of this article from the Starts With A Bang archives!