How science can solve the mystery of why we exist at all
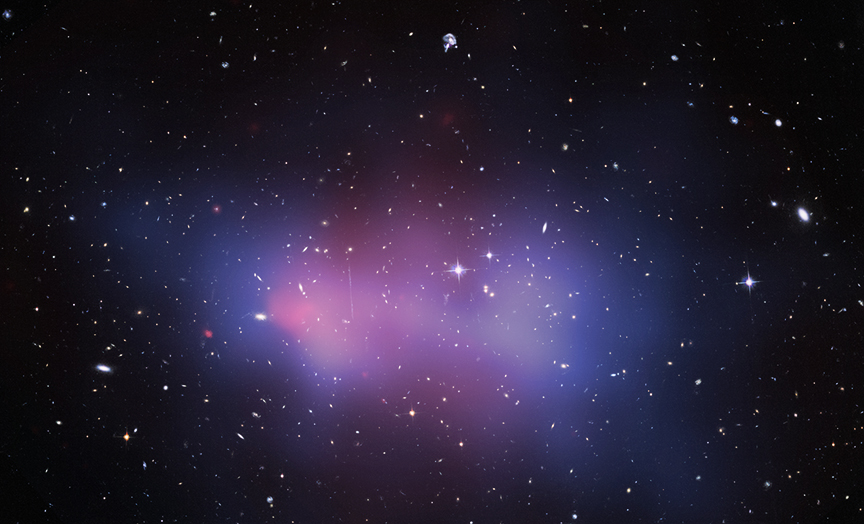
- In every experiment ever performed, including both on Earth and in the larger Universe, we can only create or destroy matter by creating or destroying an equal amount of antimatter.
- And yet, our Universe is overwhelmingly made of matter, with only a minuscule fraction-of-a-percent existing in the form of antimatter.
- Although this puzzle, known as the baryogenesis problem, remains one of the greatest unsolved mysteries in physics, we might be on the cusp of finally understanding why.
After more than 13 billion years of cosmic evolution, on a planet orbiting a rather unremarkable star in a common, run-of-the-mill galaxy, human beings came into existence for the first time. Hundreds of thousands of years later, we began to unravel many of the mysteries of the Universe. On cosmic scales, we’ve discovered that the Universe is filled with planets, stars, galaxies, and more, extending to the limits set only by the speed of light and the Big Bang. On smaller scales, meanwhile, we have unveiled the building blocks of the Universe, discovering molecules, atoms, and the subatomic particles that fundamentally compose our reality.
Yet, everywhere we look, we see the same thing: the material that makes up every planet, star, galaxy, and cloud of gas or dust is composed of matter. This is a big deal, because as far as we can tell, the only way to create or destroy matter is to create or destroy, along with it, an equal amount of antimatter. Somehow, the Universe is in a matter-dominated cosmic imbalance. This is one of the great unsolved problems of existence: the baryogenesis puzzle, or why there’s a matter-antimatter asymmetry. Although we haven’t uncovered the solution to this puzzle, there’s an awful lot we do know about it. Here’s where the science is today.

Credit: David A. Aguilar (main); NASA/GSFC/Fermi (inset)
What is the Universe made of?
The first step in solving any problem is making sure it’s actually the problem you think it is. Whenever matter and antimatter meet in the Universe, they annihilate, and matter-antimatter annihilation produces a very specific signal. When a particle of matter collides with its antimatter counterpart, it typically results in the production of two photons (in the center-of-momentum frame of reference of the collision) with equal energies and opposite momenta. An electron annihilating with a positron, for instance, produces two photons of precisely 511,000 electron-Volts of energy apiece: the energy equivalent of the mass of the particles that annihilated, via Einstein’s E = mc².
We can see these annihilation signals throughout space wherever they occur, enabling us to identify where matter and antimatter meet. If there were:
- planets,
- stars,
- galaxies,
- clusters of galaxies,
- or even intergalactic regions of space,
where some were matter and some were antimatter, we’d see evidence of those high-energy photons from annihilation at the interface. The fact that we do see those photons, but so rarely and only in specific locations (mostly consistent with emissions from active black holes), tells us that the entire observable Universe is made primarily of matter and not antimatter.
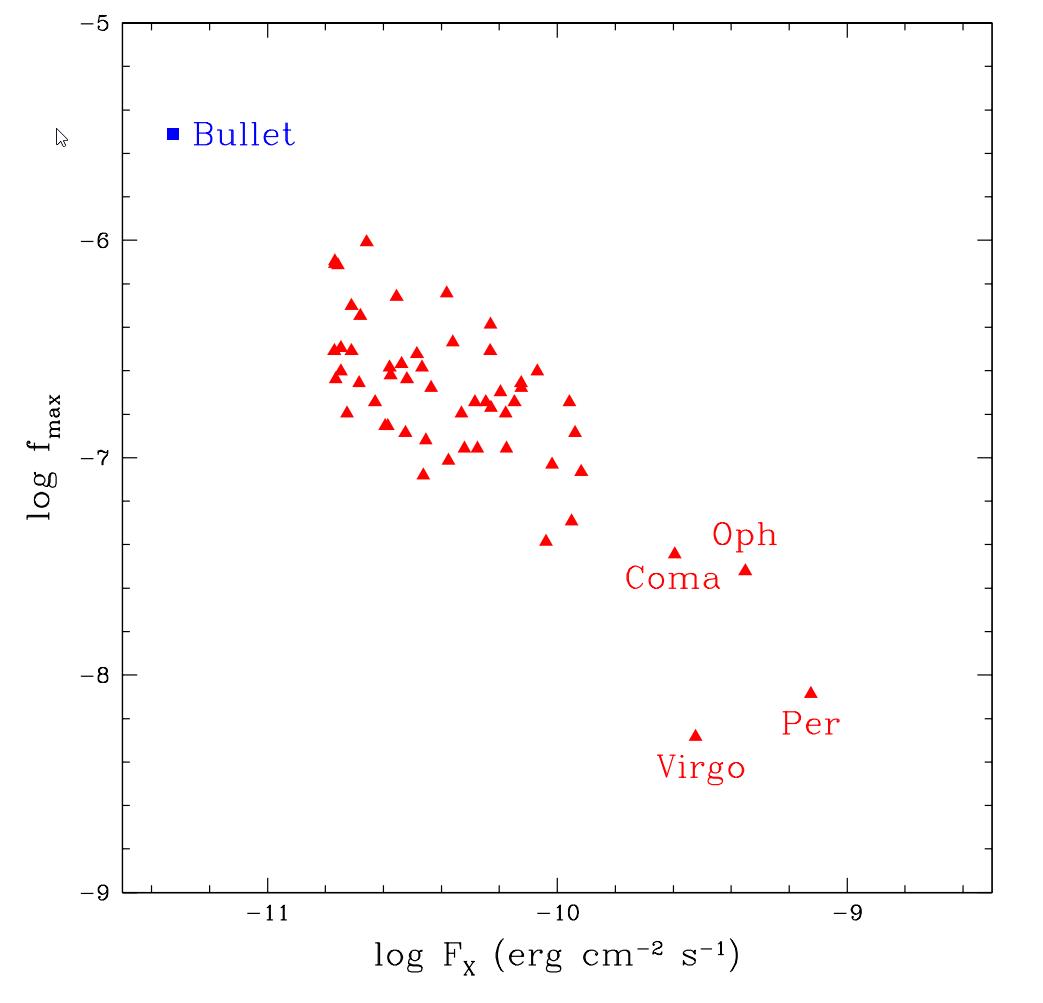
Credit: G. Steigman, JCAP, 2008
There’s also another line of evidence we can look to in order to determine what the Universe is made of: the specific ratios of the lightest elements and their isotopes in the Universe. Back before any stars formed in the Universe, in the earlier stages of the hot Big Bang, the Universe was smaller, denser, more uniform, and filled with higher-energy radiation. There’s radiation in the Universe today left over from the hot Big Bang, but it’s diffuse and low in energy; we can measure it and assign it a temperature, and we find that it’s only at 2.725 K today.
Since the energy of each light wave is defined by its wavelength, and the Universe expands over time, the wavelength of each photon gets stretched as time marches forward. If we extrapolate backwards instead, however, we find that the wavelength of each photon was shorter — more compressed — in the past, meaning that the farther back we look in time, the hotter the Universe was back in those early stages. At some point, the Universe was so hot that neutral atoms were an impossibility to form, as there were not enough photons of sufficient energy to prevent electrons from stably binding to the atomic nuclei that were present. But if we like, we can go back even farther than that.

Credit: E. Siegel/Beyond the Galaxy
We can go all the way back to an epoch where the Universe was so hot that even atomic nuclei couldn’t bind together. Every time they would attempt to do so, a photon would blast the individual protons and neutrons apart, preventing them from building up to heavier elements. Only when the Universe has cooled below a certain critical threshold — which occurs about 3 to 4 minutes after the start of the hot Big Bang — can we then start forming atomic nuclei that are heavier than a single, simple proton.
Once that moment arrives, we can build up the lightest elements in the Universe according to the rules of nuclear physics. Remarkably, the ratio of the light elements and their isotopes that we get out, including:
- hydrogen (a single proton),
- deuterium (a proton plus a neutron),
- helium-3 (two protons plus a neutron),
- helium-4 (two protons and two neutrons), and
- lithium-7 (four protons and three neutrons),
depends on only one parameter: the ratio of photons to the total number of protons and neutrons combined. When we take observations, both from the most pristine clouds of gas we can find and also from the imprint in the cosmic microwave background, we get the same answer: there’s about 1 proton or neutron for every 1.6 billion photons in the Universe. Even in the very early stages of the hot Big Bang, there was more matter than antimatter.

Credit: E. Siegel/Beyond the Galaxy (L); NASA/WMAP Science Team (R)
Why the problem is so problematic
This is a real conundrum. On the one hand, this is a good thing. If there were equal amounts of matter and antimatter in the Universe, almost all of it would have annihilated away. At present, there would be less than one particle of either matter or antimatter per cubic kilometer in the Universe remaining.
As it stands, however, the Universe is much denser than that by about a factor of a billion, and practically all of what remains is matter, not antimatter. But the only way we know of to convert energy into mass or to convert mass into energy always has the same result: the number of matter particles minus the number of antimatter particles is always a constant.
Somehow, there has to be something else going on with the particles in the Universe — beyond what the Standard Model predicts — to create the Universe as we observe it to be today. If we approach the problem scientifically, that means extrapolating back to the earliest state of the hot Big Bang, where particles and antiparticles of all types could easily be created at the highest energies, and to see what it would take for the Universe to create a matter-antimatter asymmetry where there was none initially.
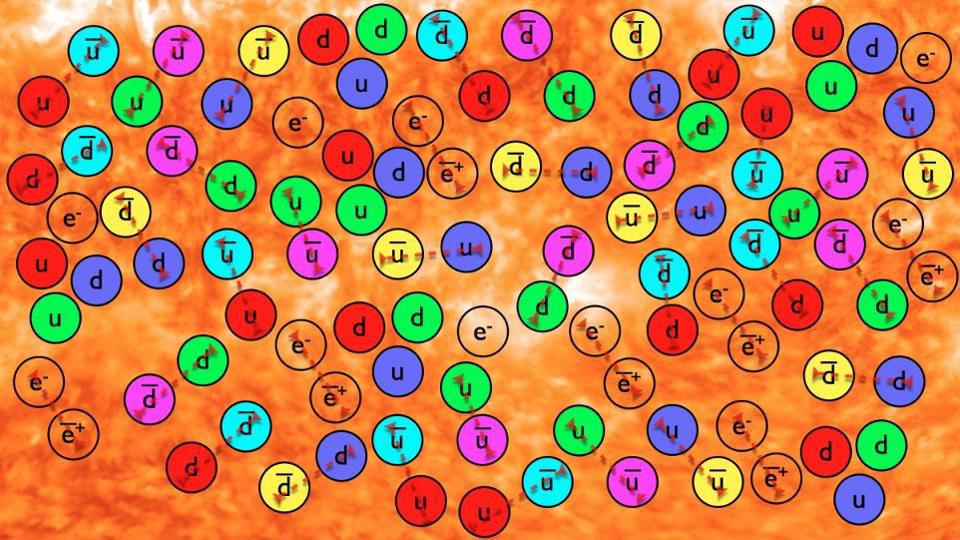
Credit: E. Siegel/Beyond the Galaxy
Every interaction between particles (and antiparticles) that we’ve ever observed, at all energies, has never created nor destroyed even a single particle of matter without also creating or destroying an equal number of antimatter particles. This is true for all quarks and antiquarks, for all leptons and antileptons, for all three of the lepton families (electron, mu, and tau), and for all combinations of these classes of particles. We do not know how to create matter without creating an equal amount of antimatter, and we do not know how to destroy antimatter without destroying an equal amount of matter.
And yet, it is theoretically possible to create a matter-antimatter asymmetry where none existed initially. Moreover, it was shown by Soviet physicist Andrei Sakharov, back in 1967, that it can be done even without violating the laws of physics. All you need to do is meet the following three criteria:
- The Universe must be out of thermal equilibrium.
- The Universe must contain examples of both C-symmetry and CP-symmetry violation.
- And the Universe must admit interactions that violate the conservation of baryon number.
These three parts, today, are known as the Sakharov conditions. (And yes, we’ll explain what they mean in plain English!) If you meet all three of these criteria, then creating a matter-antimatter asymmetry is inevitable. Here’s how it would work.

Credit: Brookhaven National Laboratory
How to solve the puzzle, theoretically
In the beginning, because you have so much energy, you will abundantly and spontaneously create all of the particles and antiparticles that energy allows you to: once again via E = mc². You’ll create them in equal numbers, but then as the Universe expands, it cools.
This is where the first step — the “out of thermal equilibrium” step — comes in. If you’re in thermal equilibrium, then the number of particles and antiparticles of each species that you create will equal the number of particles and antiparticles of that species that are destroyed with each time interval that goes by. But if you’re out of thermal equilibrium, which occurs when the Universe cools down below the energy threshold needed to create that species via E = mc², you’re out of thermal equilibrium.
When you’re out of thermal equilibrium, you create particles and antiparticles of each species more slowly than you destroy them through matter-antimatter annihilation. If the particles (and antiparticles) of that species are unstable, then when their densities drop low enough, they’ll decay rather than annihilate. And if they decay with the right properties (i.e., if they meet the other two Sakharov conditions), then they’ll have a chance to create a matter-antimatter asymmetry.

Credit: Inductiveload/Wikimedia Commons
So now let’s imagine we have unstable particles and antiparticles, and they’re going to decay. There are certain symmetries that exist between all matter and antimatter particles. For example, matter and antimatter particles all need to have the same:
- rest mass,
- magnitude of electric charge,
- quantum spin,
- allowable decay pathways,
- and total mean lifetimes
as one another. But certain properties are allowed to be different. And under the weak interactions, certain properties have already been observed to be different between matter and antimatter particles of the same species.
For example, imagine you’ve got a spinning particle, and you visualize it by curling the fingers of your left hand towards your thumb. If your thumb points upwards, then when you look “down” at your thumb, you’ll see your fingers curl in the clockwise direction. When that particle decays, it will spit a certain decay product out in the direction of your thumb a certain percent of the time.
If the Universe were perfectly symmetric between matter and antimatter, you’d expect that if you replaced that particle with an antiparticle, the corresponding decay product (the anti-version of whatever particle was spit out previously) would go in the direction of your thumb exactly the same percentage of the time.
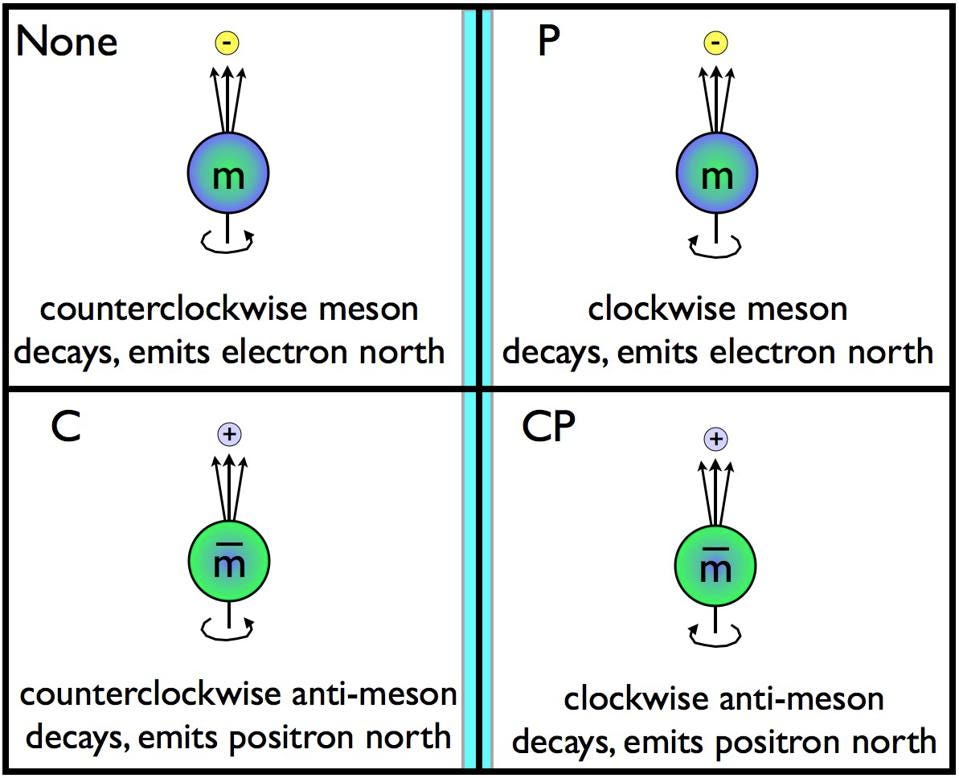
Credit: E. Siegel/Beyond the Galaxy
That’s what “C” symmetry is: replacing particles with antiparticles (and any antiparticles with their particle counterparts) and getting the same result. If you don’t get the same result, however — if you see a difference between the decays of particles and antiparticles — then that C-symmetry is violated.
Similarly, you can reflect your particles (and/or antiparticles) in the mirror: that’s “P” symmetry. If you replace particles with their mirror image counterparts and you get the same results, then you conserve P-symmetry. If you don’t get the same results, then P-symmetry is violated.
CP-symmetry is the combination of the two, meaning that to violate it, you need for your system of particles to behave differently from a system of antiparticles that has the mirror-image configuration of your own system. It’s violated in the weak interactions for decaying particles like Kaons (which contain strange quarks), as well as particles that contain bottom and charm quarks.
The only thing that hasn’t been observed is that third Sakharov condition: to see a reaction that violates the baryon number. (Each quark has a baryon number of ⅓; each antiquark has a baryon number of -⅓. No other known particles have a baryon number.) It hints to us that somewhere out there, yet undiscovered, is a reaction, interaction, or a new particle capable of producing baryon-number violating interactions.

Credit: E. Siegel/Beyond the Galaxy
So, how did the Universe actually make more matter than antimatter?
We hope that measuring particle properties intricately enough, particularly at high energies, might reveal whatever that new interaction or particle actually is. In Grand Unified Theories, for example, as illustrated above, there are new particles called X and Y bosons, and they couple to both quarks and leptons. If these particles have what are called different branching ratios from their antiparticle counterparts — meaning that the particle version decays via two different pathways with a specific ratio, while the antiparticle version takes the same corresponding pathways, but the specific ratio is different from the particle version — then all three Sakharov conditions are met, and creating a matter-antimatter asymmetry is inevitable.
New physics at the electroweak scale could also solve the baryogenesis problem. The introduction of supersymmetry is another candidate for solving the baryogenesis problem. Additionally, it’s possible there’s some interesting lepton and/or neutrino physics that appears at extremely high energies, and that could create a lepton asymmetry that the Standard Model alone could then transform into a baryon asymmetry.
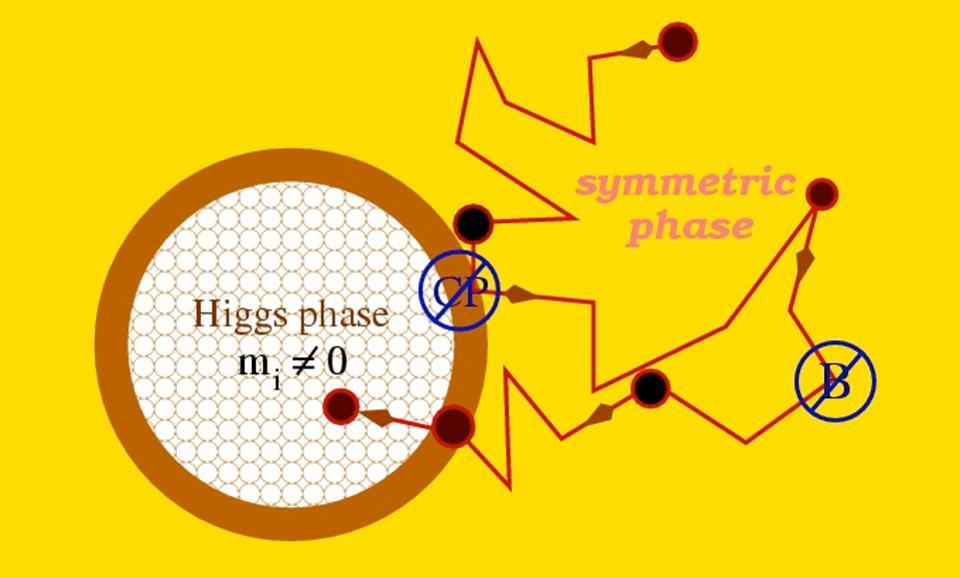
Credit: University of Heidelberg
While we don’t know exactly how the Universe came to have more matter than antimatter, we know that it was a necessary step in permitting our Universe, and the objects and creatures in it, to exist as they do. Numerous experiments from around the world are constantly probing matter and antimatter at subatomic scales, searching for any hints of baryon-number violation and for additional C-symmetry and CP-symmetry violating interactions. We might not have found the “tree of life” that allowed for our very existence, but thanks to the physics we know so far, we can be confident we’re at least looking in the right forest.